Thank you for visiting nature.com. You are using a browser version with limited support for CSS. To obtain the best experience, we recommend you use a more up to date browser (or turn off compatibility mode in Internet Explorer). In the meantime, to ensure continued support, we are displaying the site without styles and JavaScript.
- View all journals
- My Account Login
- Explore content
- About the journal
- Publish with us
- Sign up for alerts
- Open access
- Published: 19 September 2022
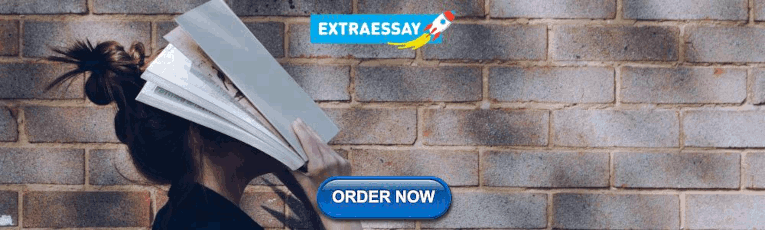
The evolutionary dynamics of extrachromosomal DNA in human cancers
- Joshua T. Lange ORCID: orcid.org/0000-0002-4246-6058 1 , 2 na1 ,
- John C. Rose ORCID: orcid.org/0000-0003-1810-4337 3 na1 ,
- Celine Y. Chen ORCID: orcid.org/0000-0002-1579-024X 4 na1 ,
- Yuriy Pichugin 5 , 6 na1 ,
- Liangqi Xie 7 , 8 ,
- Jun Tang 1 , 2 ,
- King L. Hung ORCID: orcid.org/0000-0002-3662-4662 3 ,
- Kathryn E. Yost 3 ,
- Quanming Shi 3 ,
- Marcella L. Erb 9 ,
- Utkrisht Rajkumar 10 ,
- Sihan Wu ORCID: orcid.org/0000-0001-8329-7492 11 ,
- Sabine Taschner-Mandl ORCID: orcid.org/0000-0002-1439-5301 12 ,
- Marie Bernkopf ORCID: orcid.org/0000-0001-6650-4592 12 ,
- Charles Swanton ORCID: orcid.org/0000-0002-4299-3018 13 , 14 , 15 ,
- Zhe Liu ORCID: orcid.org/0000-0002-3592-3150 7 ,
- Weini Huang ORCID: orcid.org/0000-0002-9016-2665 16 , 17 na2 ,
- Howard Y. Chang ORCID: orcid.org/0000-0002-9459-4393 3 , 18 na2 ,
- Vineet Bafna ORCID: orcid.org/0000-0002-5810-6241 10 na2 ,
- Anton G. Henssen ORCID: orcid.org/0000-0003-1534-778X 4 , 19 , 20 , 21 na2 ,
- Benjamin Werner ORCID: orcid.org/0000-0002-6857-8699 22 na2 &
- Paul S. Mischel ORCID: orcid.org/0000-0002-4560-2211 1 , 2 na2
Nature Genetics volume 54 , pages 1527–1533 ( 2022 ) Cite this article
36k Accesses
25 Citations
122 Altmetric
Metrics details
- Cell biology
- Computational biology and bioinformatics
Oncogene amplification on extrachromosomal DNA (ecDNA) is a common event, driving aggressive tumor growth, drug resistance and shorter survival. Currently, the impact of nonchromosomal oncogene inheritance—random identity by descent—is poorly understood. Also unclear is the impact of ecDNA on somatic variation and selection. Here integrating theoretical models of random segregation, unbiased image analysis, CRISPR-based ecDNA tagging with live-cell imaging and CRISPR-C, we demonstrate that random ecDNA inheritance results in extensive intratumoral ecDNA copy number heterogeneity and rapid adaptation to metabolic stress and targeted treatment. Observed ecDNAs benefit host cell survival or growth and can change within a single cell cycle. ecDNA inheritance can predict, a priori, some of the aggressive features of ecDNA-containing cancers. These properties are facilitated by the ability of ecDNA to rapidly adapt genomes in a way that is not possible through chromosomal oncogene amplification. These results show how the nonchromosomal random inheritance pattern of ecDNA contributes to poor outcomes for patients with cancer.
Similar content being viewed by others
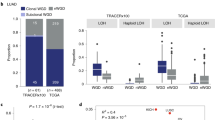
Interplay between whole-genome doubling and the accumulation of deleterious alterations in cancer evolution
Saioa López, Emilia L. Lim, … Nicholas McGranahan
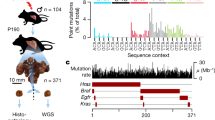
Pervasive lesion segregation shapes cancer genome evolution
Sarah J. Aitken, Craig J. Anderson, … Martin S. Taylor
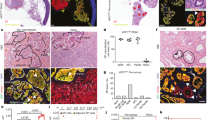
Ordered and deterministic cancer genome evolution after p53 loss
Timour Baslan, John P. Morris IV, … Scott W. Lowe
Inheritance, variation and selection are foundational principles of Darwinian organismal evolution that have been used to explain how cancers emerge, progress and adapt 1 , 2 , 3 , 4 . The concept of genetic identity by descent is central to the application of evolutionary theory to cancer, suggesting a physical basis for identity through chromosomal inheritance during cell division, thereby explaining the clonal trajectories commonly seen in tumors 5 , 6 , 7 . However, several issues challenge current models of tumor clonal evolution. First, some aggressive forms of cancer maintain high levels of intratumoral copy number heterogeneity instead of undergoing selective sweeps, as would be predicted 8 . This is especially true for amplified oncogenes, whose cell-to-cell variability is high, despite the fitness advantage conferred 9 , 10 , 11 , 12 . Consequently, the mechanisms maintaining heterogeneous oncogene amplification events have been difficult to establish. Second, the ability of some cancers to rapidly adapt to changing conditions, including treatment, by changing their genomes, especially changing the copy number of amplified oncogenes, is not well explained by current models of genetic inheritance 9 . Third, the lag time to resistance predicted by the selection for drug resistance-conferring mutations arising in a single cell, or a small number of cells, is not seen in some cancers, raising questions about whether tumors are undergoing a genetic bottleneck 9 , 13 . The presence of ecDNA amplification may explain some of these paradoxical features. Extrachromosomal oncogene amplification on circular particles that lack centromeres is now recognized as a common event in human cancer that is linked to poor outcome and treatment resistance in patients 14 , 15 . It has been suggested that ecDNAs, because they lack centromeres, are unequally segregated to daughter cells during cell division 16 , 17 , 18 . However, the impact of nonchromosomal oncogene inheritance in cancer—random identity by descent—on intratumoral genetic heterogeneity, accelerated tumor evolution, enhanced ability to withstand environmental stresses and rapid genome change on therapeutic resistance, is not well understood. In this study, we integrated computer simulations, mathematical modeling, evolutionary theory, unbiased image analysis, CRISPR-based ecDNA tagging with live-cell imaging and CRISPR-C to generate ecDNA, as well as longitudinal analyses of patients’ tumors (Extended Data Fig. 1a ), to better understand ecDNA inheritance and its functional consequences.
Random segregation of ecDNA in human cancer cells
First, we tested if different ecDNA-amplified oncogenes segregate randomly after cell division or if we can observe oncogene-specific differences. Chromosomal segregation during mitotic cell division ensures that each daughter cell has the same DNA content (Fig. 1a , red line), although in cancer, dysregulation of chromosomal segregation can also contribute to segregation errors 19 . If ecDNA segregation is completely random with equal probabilities between daughter cells to inherit ecDNA, then we predict an approximate Gaussian distribution in the per-cell content of ecDNA after mitosis (Fig. 1a and Supplementary Information 1.1 ). Therefore, we developed a FISH-based method combined with unbiased image analysis to quantify ecDNA in daughter cells after cell division, using FISH probes to detect the amplified oncogenes residing on those ecDNAs, and Aurora B kinase immunostaining to identify the daughter cells in late mitosis 20 (Fig. 1b ). In cancer cell lines of different histological types, including prostate, gastric, colon, neuroblastoma and glioblastoma, carrying different oncogenes on ecDNA, and including cancer cell lines with multiple species of oncogene-containing ecDNAs, we quantified the ecDNA distribution of approximately 100 pairs of postmitotic daughter cells per-cell line, which permits sufficient resolution (Extended Data Fig. 2a and Supplementary Information 2.3 ). These experiments revealed a wide approximate Gaussian distribution that was independent of cancer cell type or the oncogene contained on the ecDNA (Fig. 1b,c and Extended Data Fig. 2c ). The fraction of segregated ecDNA per daughter cell (histograms) was highly concordant with the theoretical prediction of random segregation (dashed line) (Kolmogorov–Smirnov test P > 0.05, Extended Data Fig. 2c ) (Fig. 1c and Methods ).
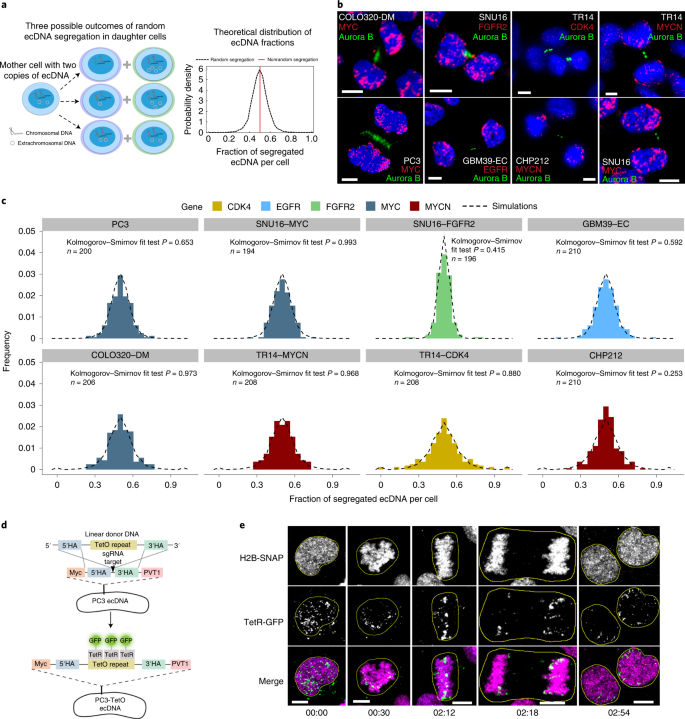
a , Schematic of ecDNA segregation and predicted distribution of ecDNA fractions. b , Representative images of ecDNA distribution to daughter cells, identified by Aurora B midbody staining, in multiple cancer cell lines in late mitosis. c , Frequency histograms of ecDNA fractions in cancer cell lines analyzed in b , showing agreement between simulated random segregations (dotted distributions) and observation (colored distributions) (Kolmogorov–Smirnov test P > 0.05). d , Schematic of the CRISPR-based genetic approach used for live-cell imaging of ecDNA in prostate cancer cells. HA, homology arms. e , Live-cell time-lapse imaging revealed unequal distribution of ecDNA between daughter cells. Time stamps, hh:mm. Scale bars, 5 μm.
Source data
To confirm these correlative observations, we designed a live-cell imaging system to visualize ecDNA dynamics during cell division. We used CRISPR–Cas9 (ref. 21 ) to insert a TetO array into the intergenic region between MYC and PVT1 of the ecDNA in PC3 prostate cancer cells (Fig. 1d ). Insertion of this array was confirmed by PCR, Sanger sequencing and TetO-MYC dual FISH (Extended Data Fig. 3a–d ). Subsequent expression of green fluorescent protein (GFP) fused to a Tet repressor, TetR-GFP, which binds the TetO array, enabled tracking of ecDNA throughout the cell cycle (Fig. 1d ). Chromatin was detected by a histone H2B-SNAP tag fusion labeled with the newly developed JF669 SNAP tag ligand 22 . Live-cell time-lapse imaging of PC3-TetO cells revealed the random inheritance pattern of ecDNA during cell division (Fig. 1e and Supplementary Video 1 ). Having demonstrated that ecDNA is randomly segregated during cell division, we investigated how ecDNA random segregation affects the other pillars of Darwinian evolution, that is, variation and selection.
ecDNA causes intratumoral heterogeneity
Intratumoral heterogeneity plays a significant role in therapy resistance and tumor evolution 23 , 24 . To better understand how random segregation of ecDNA might contribute to intratumoral heterogeneity, we performed individual-based stochastic computer simulations of growing cell populations assuming random ecDNA segregation during cell division (Supplementary Information 2.1 ). We formulated the dynamics of ecDNA per-cell distribution of ecDNA (Fig. 2a and Supplementary Information 3 ), based on the observed pattern of random segregation. Assuming independent replication and random segregation, the ecDNA dynamics can be translated into a set of coupled differential equations, where N k ( t ) denotes the number of cells with k ecDNA copies at time t and s is the coefficient of selection. The number of cells with k ecDNA then changes in time according to:
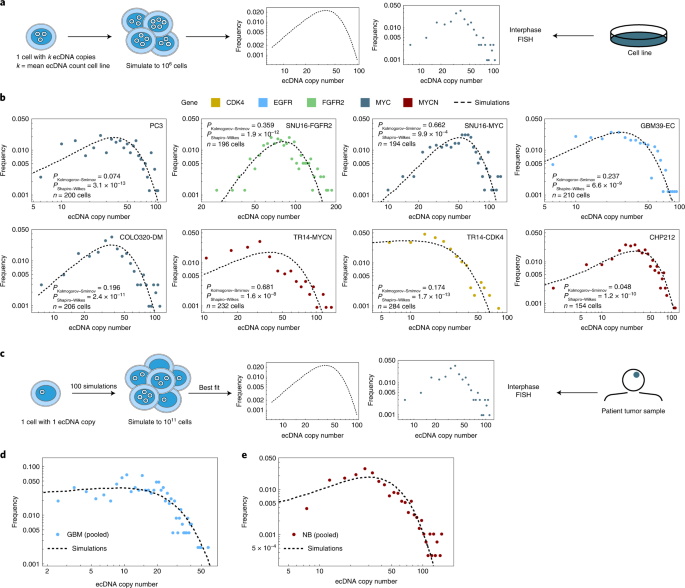
a , Schematic showing the quantification of ecDNA copy number heterogeneity from simulations of random ecDNA segregation and ecDNA + cell lines. b , ecDNA oncogene copy number measured by interphase FISH in cancer cell lines. Agreement between observed (colored histograms) and simulated (dashed histograms) revealed that oncogene copy number heterogeneity largely follows the predicted distribution. Unadjusted P values from Shapiro–Wilks and Kolmogorov–Smirnov tests are shown. c , Schematic showing the quantification of ecDNA copy number heterogeneity from simulations of random ecDNA segregation and ecDNA + patient data. d , ecDNA copy number distribution in six patients with GBM (dots) e , and four patients with NB (dots) emerges from the same process of random ecDNA segregation (black dashed line).
To make the problem computationally tractable, we utilized a Metropolis Hastings implementation of the Gillespie algorithm 25 . To mimic tumor growth, we initiated simulations with a single cell containing 1 copy of ecDNA and ran to varying population sizes up to a maximum of 10 11 cells for ecDNA under neutral ( s = 1 or positive s > 1) selection. We simulated cell line experiments by starting with 1 cell with k copies of ecDNA, where k is the mean ecDNA copy number of the cell line of interest (Supplementary Information ) and let the population grow to 10 6 cells. For these simulations, the ecDNA copy number distribution is extremely wide. Many cells are predicted to carry a few ecDNA copies and a few cells carry many (up to hundreds) of ecDNA copies. However, there is a fundamental difference in ecDNA dynamics under neutral and positive selection. If ecDNA is under positive selection, the distribution is predicted to shift toward higher copy number in time, while the distribution remains at the initial ecDNA copy number for neutral evolution.
We then compared the distributions of ecDNA copy numbers predicted by our simulations, with empirical data derived from six ecDNA + tumor lines of different cancer types with known ecDNA-amplified oncogenes. For two cancer types, glioblastoma (GBM) and neuroblastoma (NB), we had bona fide tumor tissue and clinical data available to extend our studies 9 . We also selected two cancer cell lines that had two distinct species of ecDNA as indicated (Fig. 2b ). In these cancer cell line models, the observed per-cell ecDNA copy number distributions were also very wide with extreme cell-to-cell variation that matched the distributions predicted by our simulations (Fig. 2b , Kolmogorov–Smirnov test P > 0.05 and Extended Data Fig. 4a ).
We then extended our analyses to clinical samples by quantifying the per-cell distribution of staining of an epidermal growth factor receptor (EGFR) FISH probe on tumor sections from six patients with GBM and we also quantified the per-cell distribution of a MYCN FISH probe on tumor sections from four patients with NB. Each of these patients had the amplified oncogene on ecDNA (Fig. 2c and Extended Data Fig. 5a ). Although these tissue samples were small, resulting in far fewer cells per sample, we nonetheless observed that the ecDNA copy number distributions again showed extreme cell-to-cell variation that matched the distributions predicted by our simulations (Kolmogorov–Smirnov test P > 0.05, Extended Data Fig. 5b ; Fig. 2d,e and Extended Data Fig. 5b ).
We then asked if there were indications of positive selection for ecDNA-amplified oncogenes both in our cell line data and patient samples or if the observed patterns of ecDNA heterogeneity could be explained by neutral evolution and random segregation alone. Our theoretical model makes dynamic predictions that differ for ecDNA under positive or neutral selection (Extended Data Figs. 1a and 6a ). Two major differences were the fraction of ecDNA + cells and the mean ecDNA copy number in large cell populations. In a neutral model of ecDNA evolution, fractions of ecDNA + cells decline and approach 0 in large populations, whereas the mean ecDNA copy number is time-independent and constant, for example, it will remain 1 if the population was initiated by a single cell with 1 copy of ecDNA. In contrast, for ecDNA under positive selection, the fraction of ecDNA + cells approaches 1 in large populations and the mean ecDNA copy number increases with increasing population size. Both cell line and patient data agree with models of ecDNA under positive selection (Extended Data Fig. 6b,c ). However, these observations are indirect and qualitative and a role for balancing selection over time cannot be excluded.
Drug-induced selection of ecDNA
To directly test the predictions of our model and resolve the temporal dynamics of ecDNA distribution, we set out to experimentally quantify the evolution of ecDNA from its inception. We used CRISPR-C 26 to generate ecDNAs containing the dihydrofolate reductase ( DHFR ) gene in the HAP1 cancer cell line, a near haploid chronic myelogenous leukemia human cancer cell line (Fig. 3a ). Approximately 15% of the cells contained ecDNA after CRISPR-C and each cell carrying ecDNA had exactly 1 copy. Therefore, we were able to deploy digital droplet PCR to measure how ecDNA evolved over time from its generation. The presence of a chromosomal ‘scar’ left behind after the CRISPR cutting and religation of the chromosome, enabled direct comparison of extrachromosomal and chromosomal dynamics in the same cell population. Further, by generating ecDNAs that contain the DHFR gene, we were also able to model the effects of neutral or positive selection for ecDNA, in the absence or presence, respectively, of methotrexate, which targets DHFR and interrupts nucleotide metabolism 27 . ecDNA induction is disadvantageous for cells and ecDNA copies are lost initially (Fig. 3b ). In the absence of methotrexate, after the initial selection of cells that can tolerate and maintain ecDNA copies, the mean ecDNA copy number stayed constant, in line with neutral selection as predicted by our model (Fig. 3c ). The chromosomal scar frequency also stayed constant throughout the experiment, which is consistent with neutral selection (Fig. 3d ). In contrast, we observed a strong, dose-dependent rise in ecDNA copy number in response to methotrexate treatment (Fig. 3e ), which was highly consistent with simulations of varying positive selection strengths and provided clear evidence for a strong selective advantage for cells containing DHFR ecDNA to overcome methotrexate treatment (Fig. 3f ).
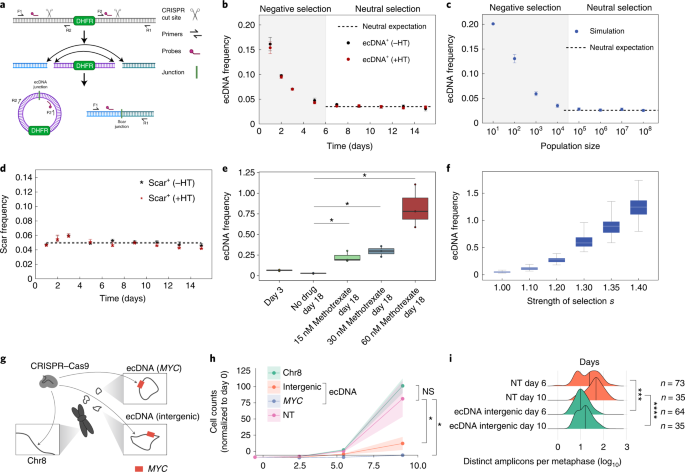
a , Schematic depicting the CRISPR-C strategy used to generate a single ecDNA in HAP1 cells containing the DHFR gene. DHFR ecDNA and the chromosomal scar are detected by ddPCR across the new junction sites. b , Tracking mean ecDNA copy number in HAP1 cells by ddPCR after day 0 induction of ecDNA by CRISPR-C. Neutral selection for DHFR ecDNA observed by similarity between hypoxanthine and thymidine omission or inclusion. c, Simulation of mean ecDNA number mimicking the experimental conditions in b . Negative selection s = 0.5, neutral selection s = 1. d , Mean frequency of the chromosomal scar determined by ddPCR across the scar junction. e , Mean ecDNA copy number after ecDNA induction on day 0 ± methotrexate treatment begun on day 4. b – e , CRISPR-C data from 3 biological replicates; data are presented as mean ± s.e.m.; P values from two-sided t -tests. e , f , Box plots are shown with line at the median and box ranging from the 25th to the 75th percentile, with the whiskers extending to the most extreme value. f , Simulation of mean ecDNA copy number mimicking the experiment in e . Negative selection s = 0.5 for 4 d followed by varying levels of selection strength as indicated for 14 d. Box plots are shown with line at the median and box ranging from the 25th to the 75th percentile, with whiskers extending to the most extreme value. g , Depiction of CRISPR-based strategy to test selective advantage given to COLO320-DM cells by MYC ecDNA. The arrows indicate regions targeted by sgRNA. h , Genome editing of MYC encoded on ecDNA caused massive decrease in cell numbers that exceeded the impact of intergenic ecDNA editing, which is indicative of strong selection for oncogenes on ecDNA. Data shown as the mean ± s.d. with P values from two-sided t -tests; data from two independent replicates. NS, not significant; NT, nontransfected. i , Quantification of ecDNA numbers per metaphase at 6 and 10 d after CRISPR transfection. Data shown with the median marked with vertical lines, P values from Mann–Whitney U -tests. * P ≤ 0.05; ** P ≤ 0.005; *** P ≤ 0.0005; **** P ≤ 0.00005.
To further analyze whether oncogene-bearing ecDNAs are under positive selection, we deployed guide RNAs targeting different genomic regions of COLO320-DM MYC ecDNA (intergenic region on ecDNA and MYC gene body on ecDNA) and a nonamplified, intergenic region of chromosome 8 (Fig. 3g ). We infected the cells with Cas9 and the single-guide RNAs (sgRNAs) by lentiviral vectors, quantifying cell proliferation and ecDNA copy number. While Cas9-targeted cutting of chromosome 8 showed minimal impact on cell proliferation, targeting of the ecDNA on an intergenic region, and even more so on MYC on the ecDNA, caused an extreme growth deficit (Fig. 3h ). When we quantified ecDNA copy number in these cells, we saw a significant decrease in ecDNA 6 d after initial infection (Fig. 3i and Extended Data Fig. 6d ). Taken together, these CRISPR-C data (Fig. 3a–f ) confirm that ecDNAs, and the oncogenes contained therein, are under strong selective pressure, which influences the mean ecDNA oncogene copy number and per-cell distribution in tumors. Correlative analyses of ecDNA copy number in the cell line models and tumor samples were highly consistent with our simulations and with our model for strong positive selection of oncogene-bearing ecDNA in tumors (Extended Data Figs. 1 and 6c ). However, open questions remain. As additional data and higher single-cell resolution are achieved in the near future, it will be important to explore a potential role for balancing or other forms of copy number-dependent selection of ecDNA in patients with cancer and determine the forces that shape these processes. Mapping the time-resolved ecDNA copy number distribution at single-cell resolution for different ecDNA-amplified oncogenes will be critical future work to better understand more complex fitness models.
ecDNAs afford rapid tumor adaptation to stress
Having shown that ecDNA contributes to each of the three pillars of Darwinian evolution—inheritance (that is, random identity by descent), variation and selection—in a unique fashion relative to chromosomal inheritance, we asked whether these ecDNA features enable more rapid tumor adaptation to stress than possible through chromosomal inheritance (Fig. 4a ). We utilized an isogenic cell line pair derived from a patient with GBM 9 to examine the importance of ecDNA in driving rapid adaptation. GBM39-EC is a patient-derived neurosphere model with a mean copy number of approximately 100 copies of EGFRvIII , a gain-of-function EGFR mutation residing on ecDNA 15 , 28 . GBM39-homogeneously staining region (HSR) is an isogenic model, in which all the EGFRvIII amplicons reside on chromosomal HSRs, at the same mean copy number with the same DNA sequence (Extended Data Fig. 7a ) 28 . Importantly, the heterogeneity of EGFRvIII copy number in GBM39-EC correlates with the heterogeneity of EGFRvIII protein expression assessed by flow cytometry (Extended Data Fig. 7b,c ). GBM39-EC cells are highly glycolytic 9 . Therefore, we tested the differential effect of glucose restriction on GBM39-EC and GBM39-HSR cells. We withdrew 80% of normal glucose levels from the culture medium and saw a striking difference—the GBM39-HSR cells were exquisitely sensitive to glucose withdrawal whereas the GBM39-EC cells showed no significant decrease in cell growth (Fig. 4b ). This ability of GBM39-EC cells to adapt to glucose restriction was mirrored by a rapid decrease in the mean level and overall distribution of EGFRvIII -containing ecDNAs per cell (Fig. 4c ). Remarkably, this genomic shift took place within a couple of cell cycles. In contrast, the GBM39-HSR cells, which were more homogeneous with respect to EGFRvIII copy number, were highly sensitive to glucose restriction (Fig. 4c ).
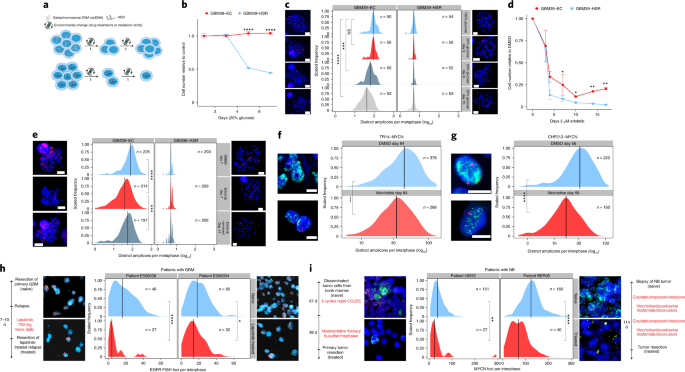
a , Schematic depicting how the random segregation of ecDNA and ensuing heterogeneity can drive rapid adaptation and resistance. b , ecDNA-containing GBM cells were resistant to glucose withdrawal, whereas GBM cells in which the same oncogene had lodged onto chromosomal loci at near identical copy number (GBM39-HSRs) did not tolerate glucose withdrawal; data from three independent replicates; presented as the mean ± s.d. c , Adaptation of ecDNA-containing cells to glucose withdrawal was linked to a rapid shift in the distribution of amplicons per cell, unlike the highly sensitive HSR-containing cells, which did. not modulate amplicon copy number. The timeline of the experiment is depicted on the left. The red FISH signal was from the EGFR FISH probe. d , GBM cells with EGFRvIII amplified on ecDNA, after an initial response, rapidly became resistant to the EGFR tyrosine kinase inhibitor erlotinib, whereas GBM39-HSR cells remained highly sensitive. Data are presented as mean ± s.d.; data from 2 independent replicates (day 7 from 4 replicates). e , GBM cells with EGFRvIII amplified on ecDNA rapidly shifted the distribution of EGFRvIII amplicons per cell, measured at 7 d, which can also be rapidly reversed within 1 week by drug withdrawal. The timeline of the experiment is depicted on the left. The red signal is the EGFR FISH probe. f , The NB cell line TR14 shifted the copy number distribution of MYCN ecDNA when treated with 43 nM vincristine for 12 weeks. g , The NB cell line CHP212 shifted the copy number distribution of MYCN ecDNA when treated with 5.3 nM vincristine for 8 weeks. h , Comparison of the distribution of EGFR amplification per cell in two patients with GBM before therapy (naive) and after 7–10 d of lapatinib treatment. The red FISH signal is from the EGFR FISH probe. The green FISH signal is from the Chr. 7 control probe. i , Comparison of MYCN ecDNA copy numbers assessed by MYCN (green) FISH in two patients with NB before and after receiving chemotherapy including vincristine. The red signal is from the Chr. 2 control FISH probe. Scale bar, 5 μm; scale information was not available for clinical tissue images. P values were calculated using a Mann–Whitney U -test for comparisons of distributions and two-sided t -tests for comparisons of cell numbers. * P ≤ 0.05; ** P ≤ 0.005; *** P ≤ 0.0005; **** P ≤ 0.0005.
We had previously shown that GBM39-EC cells could become reversibly resistant to the EGFR tyrosine kinase inhibitor erlotinib by lowering ecDNA copy number 9 . Therefore, we examined whether GBM39-EC cells would develop resistance to erlotinib more rapidly than GBM39-HSR cells. Like glucose deprivation, GBM39-EC adapted to the changing condition by altering its ecDNA copy number. After initially decreasing in cell number, GBM39-EC cells became resistant to erlotinib after just two weeks of treatment, shifting their per-cell ecDNA distribution in a reversible fashion (Fig. 4d,e and Extended Data Fig. 7d ). In contrast, the GBM39-HSR cells did not shift EGFRvIII chromosomal copy number and remained highly sensitive to erlotinib (Fig. 4d,e and Extended Data Fig. 7f ). We then analyzed two samples taken from the tumors of patients with GBM, as described previously 2 . We compared the primary tumor resection (naive) to the resected relapse, which was treated with EGFR tyrosine kinase inhibitor lapatinib for 7–10 d before resection. We found a significant decrease in mean EGFR copy number and in the ecDNA distribution in the tumors of these patients (Fig. 4h ). To extend our analysis to other ecDNA-containing cancer types, we studied the effect of vincristine, a chemotherapeutic that antagonizes MYCN amplification 29 . In vitro, in the NB cell lines TR14 and CHP212 with MYCN amplified on ecDNA, tumor cells with lower copy number were selected in response to vincristine (Fig. 4f, g ). When we compared treatment-naive NB biopsies with primary tumor resections after treatment including vincristine, we found a similarly significant decrease in the mean copy number and a shift in the ecDNA distribution of MYCN to a lower copy number in both of the tumors of these patients, in parallel with the cell line data (Fig. 4i ). Interestingly, when TR14 cells were treated with the CDK4/6 inhibitors abemaciclib, and to a greater extent palbociclib, a shift in the distribution of CDK4 ecDNA to higher copy number was detected in resistant tumor cells (Extended Data Figs. 7d,e and 8 ), which is consistent with previous reports across different tumors showing that high CDK4 copy number and expression promotes resistance to CDK4/6 inhibitors 30 .
Together, these data indicate a clear pattern of how ecDNA enables high levels of heterogeneity, which enable increased initial resistance to environmental or therapeutic challenges. Further, the ongoing random inheritance of ecDNA-based oncogenes allows rapid adaptation and the formation of resistance through a mechanism that is impossible in cells driven by chromosomal alterations.
ecDNA has emerged as a major challenge that forces reconsideration of our basic understanding of cancer. Emerging data demonstrate that the altered topology of ecDNA drives enhanced chromatin accessibility and rewires gene regulation to drive oncogenic transcription 28 . Further, the unique higher-level organization of ecDNA particles into hubs 31 further contributes to ecDNA-mediated pathogenesis. The findings presented in this article reveal that ecDNA uniquely shapes each of the foundational principles of Darwinian evolution, that is, random inheritance by descent, enhanced variation through random segregation and selection, thereby accelerating tumor cell evolution and increasing adaptability. Such observations may explain why clinical activity from therapies targeting oncogenic amplification events are so limited in tumors such as GBM where ecDNAs are so prevalent. Treating such cancers may require targeting the unique adaptability of ecDNAs in the future.
Our research complies with all relevant ethical guidelines. FISH images from GBMs were obtained from patients treated at UCLA participating in a multi-institutional phase II clinical trial of lapatinib sponsored by the North American Brain Tumor Consortium NABTC 04-01, a biomarker and phase II study of lapatinib GW572016 (lapatinib) in recurrent GBM. The collection and use of patient samples was approved by the UCLA institutional review board. These samples have been described previously, including in Nathanson et al. 9 . FISH images from NBs were acquired as part of routine molecular tumor diagnostics. Patients were registered and treated according to the trial protocols of the Society of Paediatric Oncology European Neuroblastoma Network HR-NBL-1 trial (NCT01704716) or the German Society of Pediatric Oncology and Hematology (GPOH) NB2004 trial. This study was conducted in accordance with the World Medical Association Declaration of Helsinki (2013) and good clinical practice; informed consent was obtained from all patients or their guardians. The collection and use of patient specimens was approved by the institutional review boards of the St. Anna Kinderspital in Vienna, the Charité-Universitätsmedizin Berlin and the Medical Faculty, University of Cologne. Specimens and clinical data were archived and made available by Charité-Universitätsmedizin Berlin, the St. Anna Kinderspital or the National Neuroblastoma Biobank and Neuroblastoma Trial Registry (University Children’s Hospital Cologne) of the GPOH.
Cell culture
Cell lines were purchased from ATCC or the DSMZ-German Collection of Microorganisms and Cell Cultures (Leibniz Institute) or were a kind gift from J.H. Schulte. GBM39-HSR and GBM39-EC were derived from a patient with GBM as described previously 9 . Hap1 cells (Horizon Discovery) were maintained in IMDM supplemented with GlutaMAX and 10% FCS (Gibco).
PC3 cells were cultured in DMEM with 10% FCS. COLO320-HSR and COLO320-DM were cultured in DMEM/F12 50:50% with 10% FCS. SNU16 were grown in Roswell Park Memorial Institute (RPMI) 1640 with 10% FCS. GBM39-HSR and GBM39-EC neurospheres were grown in DMEM/F12 with B27, GlutaMAX, heparin (5 μg ml −1 ), EGF (20 ng ml −1 ), and fibroblast growth factor (20 ng ml −1 ). TR14 cells were grown in RPMI 1640 with 20% FCS. Cell numbers were counted with a TC20 automated cell counter (Bio-Rad Laboratories). For drug treatments, the drug was replaced every 3–4 d.
Metaphase chromosome spreads
Cells were concentrated in metaphase by treatment with KaryoMAX Colcemid (Gibco) at 100 ng ml −1 for between 3 h and overnight (depending on the cell cycle speed). Cells were washed once with PBS and a single-cell suspension was incubated in 75 mM KCl for 15 min at 37 °C. Cells were then fixed with Carnoy’s fixative (3:1 methanol:glacial acetic acid) and spun down. Cells were washed with fixative three additional times. Cells were then dropped onto humidified glass slides.
Fixed samples on coverslips or slides were equilibrated briefly in 2× SSC buffer. They were then dehydrated in ascending ethanol concentrations of 70, 85 and 100% for approximately 2 min each. FISH probes were diluted in hybridization buffer (Empire Genomics) and added to the sample with the addition of a coverslip or slide. Samples were denatured at 72 °C for 2 min and then hybridized at 37 °C overnight in a humid and dark chamber. Samples were then washed with 0.4× SSC then 2× SSC 0.1% Tween 20 (all washes lasting approximately 2 min). 4,6-Diamidino-2-phenylindole (DAPI) (100 ng ml −1 ) was applied to samples for 10 min. Samples were then washed again with 2× SSC 0.1% Tween 20 then 2× SSC. Samples were briefly washed in double-distilled H 2 O and mounted with ProLong Gold. Slides were sealed with nail polish.
Dual immunofluorescence–FISH
Asynchronous cells were grown on poly-L-lysine-coated coverslips (laminin for GBM39-EC). Cells were washed once with PBS and fixed with cold 4% paraformaldehyde (PFA) at room temperature for 10–15 min. Samples were permeabilized with 0.5% Triton X-100 in PBS for 10 min at room temperature and then washed with PBS. Samples were then blocked with 3% BSA in PBS 0.05% Triton X-100 for 30 min at room temperature. Samples were incubated in primary antibody, diluted in blocking buffer (1:100–1:200) for either 1 h at room temperature or overnight at 4 °C. Samples were washed three times in PBS 0.05% Triton X-100. Samples were incubated in secondary antibody, diluted in blocking buffer for 1 h at room temperature (all subsequent steps in the dark) and then washed three times in PBS 0.05% Triton X-100. Cells were washed once with PBS and refixed with cold 4% PFA for 20 min at room temperature. Cells were washed once with PBS then once with 2× SSC buffer. FISH proceeded as described above with the following difference: denaturation was performed at 80 °C for 20 min.
Conventional fluorescence microscopy was performed using an Olympus BX43 microscope; images were acquired with a QIClick cooled camera. Confocal microscopy was performed using a Leica SP8 microscope with lightning deconvolution (University of California San Diego School of Medicine Microscopy Core). NB cell lines were imaged with a Leica TCS SP5 microscope, HCC PL APO lambda blue ×63 1.4 oil lens or with DeltaVision Elite Cell Imaging System (Applied Precision) and microscope (model IX-71; Olympus) controlled by the SoftWoRx software v.6.5.2 (Applied Precision) and a 60x objective lens with a CoolSNAP HQ2 camera (Photometrics).
NB patient tissue FISH
FISH analysis was performed on 4-µm sections of formalin-fixed, paraffin-embedded blocks. Slides were deparaffinized, dehydrated and incubated in pretreatment solution (Dako) for 10 min at 95–99 °C. Samples were treated with pepsin solution for 2 min at 37 °C. For hybridization, the Zyto Light SPEC MYCN /2q11 Dual Color Probe (ZytoVision ) was used. Incubation took place overnight at 37 °C, followed by counterstaining with DAPI. For each case, signals were counted in 50 nonoverlapping tumor cells using a fluorescence microscope (BX63 Automated Fluorescence Microscope; Olympus). Computer-based documentation and image analysis was performed with the SoloWeb Imaging System (BioView Ltd) MYCN amplification ( MYCN FISH + ) was defined as an MYCN /2q11.2 ratio >4.0, as described in the INRG report 32 .
Quantification of FISH foci
Quantification of FISH foci was performed using the ImageJ Find plugin maxima function in a supervised fashion. To quantify pixel intensity, the ImageJ Pixel intensity function was used. The FISH images of the tissue of these two patients with GBM were obtained as part of a phase II lapatinib GBM clinical trial described previously. In brief, patients were administered 750 mg of lapatinib orally twice a day for 7–10 days (depending on whether the treatment interval fell over a weekend) before surgery, the time to steady state. Blood and tissue samples were obtained at the time of resection 9 .
Construction of PC3-TetO cell line
The insertion of TetO repeats was conducted through CRISPR–cas9-mediated approaches. The plasmids pSP2-96-mer TetO-EFS-BlaR and F9-TetR-EGFP-IRES-PuroR used in this were kind gifts from H. Zhao 21 . Briefly, the intergenic region between MYC and PVT1 was selected as the insertion region on the basis that it is amplified in PC3 cells on ecDNA with high frequency. DNA sequences were retrieved from the UCSC Genome Brower; repetitive and low complexity DNA sequences were annotated and masked by RepeatMasker in the UCSC Genome Browser. The guide sequences of sgRNAs were designed by the CRISPRdirect web tool 33 and their amplification was confirmed with whole-genome sequencing data. The guide sequence selected was constructed into pSpCas9(BB)-2A-Puro (PX459). pSpCas9(BB)-2A-Puro(PX459) was a gift from F. Zhang (Addgene plasmid no. 62988; http://n2t.net/addgene:62988 ; research resource identifier: Addgene_62988). The repair donor was obtained through PCR amplification, using the pSP2-96-merTetO-EFS-BlaR plasmid as template, as well as primers containing the 50-nucleotide homology arm upstream and downstream of the predicted cutting site.
The transfection of the CRISPR–Cas9 plasmid and 96-mer TetO EGFP-BlastR donor into PC3 cells was conducted with the X-tremeGENE HP transfection reagent according to the manufacturer’s instructions with the CRISPR–Cas9 plasmid only or the 96-mer TetO EGFP-BlastR only used as negative control. Two days after transfection, blasticidin was added to the culture medium for 3 d, at a time point when most of the cells in the negative control groups had died while more cells survived in the group with transfection of the CRISPR–Cas9 plasmid and donor. The surviving cells were subjected to limited dilution in a 96-well plate, with blasticidin being added all the time. Surviving clones were expanded and their genomic DNA (gDNA) was extracted and subjected to genotyping with a pair of primers flanking the inserted region. The PCR product of the genotyping results was subjected to Sanger sequencing to confirm the insertion at the predicted cutting site. Clones with positive genotyping bands were expanded and metaphase cells were collected. Double FISH with FISH probe against the Tet operator and against the MYC FISH probe was performed on the metaphase spread. PC3 cells with TetO repeats were infected with lentivirus containing F9-TetR-EGFP-IRES-PuroR; 2 d after infection, puromycin was added to the culture medium to establish a stable cell line that could be used to image ecDNA with the aid of EGFP visualization.
Live-cell imaging of ecDNA
The PC3 TetO TetR-GFP cell line was transfected with a PiggyBac vector expressing H2B-SNAPf and the super PiggyBac transposase (2:1 ratio) as described previously 34 . Stable transfectants were selected by 500 µg ml −1 G418 and sorted by flow cytometry. To facilitate long-term time-lapse imaging, 10 µg ml −1 human fibronectin was coated in each well of an 8-well Lab-Tek chambered cover glass. Before imaging, cells were stained with 25 nM SNAP tag ligand JF 669 (ref. 22 ) at 37 °C for 30 min followed by 3 washes with regular medium for 30 min in total. Cells were then transferred to an imaging buffer containing 20% serum in 1× Opti-Klear live-cell imaging buffer at 37 °C. Cells were imaged on a Zeiss LSM880 microscope prestabilized at 37 °C for 2 h. We illuminated the sample with a 1.5% 488-nm laser and 0.75% 633-nm laser with the EC Plan-Neofluar ×40/1.30 oil lens, beam splitter MBS 488/561/633 and filters BP 495–550 + LP 570. Z-stacks were acquired with a 0.3-µm z step size with 4-min intervals between each volumetric imaging for a total of 16 h.
Colony formation assay
TR14 cells were taken from 60 d of treatment with either dimethylsulfoxide (DMSO), 50 nM palbociclib or 5 nM abemaciclib, and seeded into a poly-D-lysine-coated 24-well plate at 20,000 cells per well. After 24 h, the cells from each condition were treated with either DMSO, 50 nM palbociclib or 5 nM abemaciclib over 20 d in triplicate. At 20 d, crystal violet staining was performed. Briefly, the cell culture medium was aspirated, cells were washed gently with PBS, fixed in 4% PFA in PBS for 20 min, stained with 2 ml crystal violet solution (50 mg in 50 ml 10% ethanol in Milli-Q water), washed once with PBS and dried for 30 min. The area intensity was calculated using the ColonyArea plugin in ImageJ v2 (NIH) 35 .
CellTiter-Glo
TR14 cells were taken from 60 d of treatment with either DMSO, 50 nM palbociclib or 5 nM abemaciclib and seeded into white flat-bottom 96-well plates (Corning) in 100 µl medium at a density of 500 cells per well. After 24 h, cells were treated with either vehicle, 50 nM palbociclib or 5 nM abemaciclib (50 µl of drug solution per well). Cell viability was determined using the CellTiter-Glo Luminescent Cell Viability Assay (Promega Corporation) at 3, 6 and 9 d after the drug was added, according to the manufacturer’s protocol.
Flow cytometry
Single-cell suspensions were made and passed through a cell filter to ensure single-cell suspension. Cells were suspended in flow cytometry buffer (Hanks’ Balanced Salt Solution buffer without calcium and magnesium, 1× GlutaMAX, 0.5% (v/v) FCS, 10 mM HEPES). EGFRvIII monoclonal antibody 806 (ref. 36 ) was added at 1 μg per million cells and incubated on ice for 1 h. Cells were washed in flow cytometry buffer and resuspended in buffer with anti-mouse Alexa Fluor 488 antibody (1:1,000, catalog no. A11017; Thermo Fisher Scientific) for 45 min on ice in the dark. Cells were washed again with flow cytometry buffer and resuspended in flow cytometry buffer at approximately 4 million cells per milliliter. Cells were sorted using a Sony SH800 FACS sorter, which was calibrated; gating was informed using a secondary-only negative control. The sorting strategy is shown in Supplementary Fig. 14 .
Quantitative PCR
DNA extraction was performed using the NucleoSpin Tissue Kit (Macherey-Nagel) according to the manufacturer’s protocol. Quantitative PCR (qPCR) was performed using 50 ng or 1.5 µl of template DNA and 0.5 µM primers with the SYBR Green PCR Master Mix (Thermo Fisher Scientific) in FrameStar 96-well PCR plates (4titude). Reactions were run and monitored on a StepOnePlus Real-Time PCR System (Thermo Fisher Scientific) and Ct values were calculated with the StepOne Plus software v.2.3 (Thermo Fisher Scientific): CDK4 forward: AAAGTTACCACCACACCCCC; CDK4 reverse: AGTGCTAAGAAAGCGGCACT.
Guide RNA design for CRISPR-C
sgRNAs were designed to target the ends of a previously reported DHFR-containing ecDNA amplicon (clone: PD29424h) 17 ; 1,000 base pairs (bp) of sequence flanking each end of this segment (Chr5: 79,841,431–81,655,326; hg19) were used to design guides using the Integrated DNA Technologies (IDT) Custom Alt-R CRISPR–Cas9 guide RNA software ( https://www.idtdna.com/site/order/designtool/index/CRISPR_CUSTOM ). These sequences were ordered as Alt-R sgRNAs (IDT).
ecDNA induction by CRISPR-C
Hap1 cells were trypsinized, quenched with IMDM (GlutaMAX, 10% FCS), counted and centrifuged at 300 g for 5 min. Cells were washed once with PBS before resuspension in Neon Resuspension Buffer to 1.1 × 10 7 cells ml −1 . Ribonucleoprotein (RNP) complexes were formed as follows: Cas9 (IDT) was diluted to 36 μM in Neon Resuspension Buffer. Equal volumes of diluted Cas9 and sgRNA (44 μM in TE, pH 8.0) were mixed and incubated at room temperature for 10–20 min. Left (DHFR_H2_sgL) and right (DHFR_H2_sgR) sgRNA RNPs were assembled separately. Then, 5.5 μl of each RNP, 5.5 22 μl of electroporation enhancer (10.8 μM; IDT) and 99 μl of cells were mixed and electroporated according to the manufacturer’s instructions using a 100-μl Neon pipet tip and electroporated with the Neon Transfection System (Thermo Fisher Scientific) using the following settings: 1,575 V, 10-ms pulse width, 3 pulses. Single-guide controls were prepared as above except 11 μl of the appropriate sgRNA was used. Electroporated cells were dispensed into 3.2 ml of medium (± hypoxanthine and thymidine supplementation as appropriate) and split into 6 wells of a 24-well plate. Negative electroporation control cells were resuspended in Neon Resuspension Buffer and then added directly to wells containing fresh medium.
For neutral selection, cells were cultured in 24-well plates and passaged every 2 d. During passaging, 80–90% of the cells in each well were used for gDNA isolation, while the rest were transferred to a new plate containing fresh medium. For hypoxanthine- and thymidine-supplemented wells, hypoxanthine and thymidine supplement (100X, catalog no. 11067030; Gibco) was added to final concentrations of 100 μΜ hypoxanthine and 16 μΜ thymidine.
For positive selection, 3 d after electroporation, cells were passaged into 12-well plates and the day 3 time point was collected. Cells were changed to medium containing the indicated concentration of methotrexate (Calbiochem) 4 d after electroporation. Medium was changed every 2–3 days and cells were passaged when at 70–80% confluence. Cells were collected after 14 days of methotrexate incubation (18 days after electroporation). The final DMSO concentration in methotrexate-treated wells was 0.1%.
Cells were collected at the indicated time points as follows: cells were washed with 1 ml per well prewarmed PBS (Gibco), followed by the addition of 100 μl TrypLE Express (Thermo Fisher Scientific) and incubation at 37 °C for 5–10 min. TrypLE was quenched with 800 μl IMDM (GlutaMAX, 10% FCS) and the cell suspension was pelleted at 300 g for 5 min at 4 °C. The supernatant was discarded and the cell pellets were stored at −80 °C.
The CRISPR-C schematic was created with BioRender.
ddPCR to determine ecDNA or chromosomal scar frequency
gDNA was isolated using DNeasy columns (QIAGEN) according to the manufacturer’s instructions, including a 10-min incubation at 56 °C during the proteinase K digestion step; DNA was eluted with 100 μl EB buffer.
Amplicons for the ecDNA junction, chromosomal scar junction and glyceraldehyde 3-phosphate dehydrogenase (GAPDH) were designed using the IDT PrimerQuest software ( https://www.idtdna.com/PrimerQuest/Home/Index ). Dual-quenched probes (IDT) were used: FAM-labeled probes were used for both the ecDNA and chromosomal scar junction amplicons to facilitate multiplexing with the GAPDH amplicon utilizing a HEX-labeled probe. All probe and primer sequences are available in Supplementary Information . Droplets were created using droplet-generating oil for probes, DG8 cartridges, DG8 gaskets and the QX200 Droplet generator (Bio-Rad Laboratories); amplification was performed using the ddPCR Supermix for Probes (Bio-Rad Laboratories). The ddPCR Supermix amplification reactions were set up according to the manufacturer’s specifications (Bio-Rad Laboratories). Approximately 60 ng of gDNA was used in a 20 μl reaction with a final primer concentration of 900 nM (225 nM for each primer), 125 nM FAM probe and 125 nM HEX probe. The reaction was partitioned into droplets for amplification according to the manufacturer’s protocol (Bio-Rad Laboratories). Droplets were transferred to a 96-well PCR plate and heat-sealed using the PX1 PCR plate sealer (Bio-Rad Laboratories). Droplets were amplified using the following cycling conditions: 95 °C for 10 min, 40 cycles (94 °C for 30 s, 56.1 °C for 60 s), 98 °C for 10 min. After thermal cycling, droplets were scanned individually using the QX200 Droplet Digital PCR system (Bio-Rad Laboratories). Positive and negative droplets in each fluorescent channel (HEX, FAM) were distinguished on the basis of fluorescence amplitude using a global threshold set by the minimal intrinsic fluorescence signal resulting from imperfect quenching of the fluorogenic probes (negative droplets) compared to the strong fluorescence signal from cleaved probes in droplets with amplified template(s). The frequency of ecDNA or chromosomal scar was calculated by dividing their measured concentration by the concentration of the GAPDH amplicon.
Quantification of single-cell ecDNA segregation patterns
We generated the theoretically expected distribution of ecDNA copy number fractions after a single cell division under different models of ecDNA segregation by stochastic computer simulations implemented in C++. Briefly a single cell is initiated with a random number of ecDNA copies n , drawn from a uniform distribution U (20,200). EcDNA is amplified and 2 n ecDNA copies are segregated between two daughter cells after a binomial trial B (2 n , p ) with segregation probability p . In this case, p = 1/2 corresponds to random segregation and p > 1/2 to a biased random segregation. This results in two daughter cells with ecDNA copy number n 1 ≈ B (2 n , p ) and n 2 = n − n 1 . The fraction of segregated ecDNA, f , is then calculated as:
\(f_1 = \frac{{n_1}}{{n_1 + n_2}}\,{{{\mathrm{and}}}}\,f_2 = \frac{{n_2}}{{n_1 + n_2}}.\)
Iterating the process 10 7 times generates the expected distribution of f as shown in Fig. 1c . Similarly, we generated an expected distribution of f for chromosomal patterns of inheritance. For perfect chromosomal segregation, we have f 1 = f 2 = 1/2. To allow for mis-segregation, we introduced a probability u = 0.05 such that n 1 = n ± 1 and n 2 = n − n 1 . We used Kolmogorov–Smirnov statistics to compare the theoretically expected and experimentally observed distributions of ecDNA copy number fractions under these different scenarios.
Stochastic simulations of ecDNA population dynamics
We implemented individually based stochastic computer simulations of the ecDNA population dynamics in C++. For each cell, the exact number of ecDNA copies was recorded through the simulation. Cells were chosen randomly but proportional to fitness for proliferation using a Gillespie algorithm. The simulation was initiated with one cell carrying n 0 copies of ecDNA. The proliferation rate of cells without ecDNA was set to r − = 1 (time is measured in generations). A fitness effect for cells with ecDNA then corresponded to a proliferation rate r + = s. In this example, s > 1 models a fitness advantage, 0 < s < 1 a fitness disadvantage and s = 1 corresponds to no fitness difference (neutral dynamics, r + = r − ). During proliferation, the number of ecDNA copies in that cell are doubled and randomly distributed into both daughter cells according to a binomial trail B ( n , p ) with success rate p = 1/2. If a cell carries no ecDNA, no daughter cell inherits ecDNA. We terminated simulations at a specified cell population size. We output the copy number of ecDNA for each cell at the end of each simulation, which allowed us to construct other quantities of interest, such as the ecDNA copy number distribution, the time dynamics of moments, the power law scaling of tails or Shannon diversity index. We used the Kolmogorov–Smirnov statistics to test similarity between simulated and experimental ecDNA copy number distributions and Shapiro–Wilk statistics to test for deviations from normality.
Sampling and resolution limits
We ran an in silico trial to test our ability to reconstruct the true ecDNA copy number distribution from a sampled subset of varying sizes. We constructed a simulated ecDNA copy number distribution from 2 × 10 6 cells using our stochastic simulations. We then performed 500 random samples of 25, 50, 100 and 500 cells, reconstructed the sampled ecDNA copy number distribution and compared similarity to the true copy number distribution using the Kolmogorov–Smirnov statistics. The distribution converges to the true distribution with increasing sampling size and a comparably small sample of 100–500 cells is sufficient to reconstruct the true underlying ecDNA copy number distribution.
Mathematical description of ecDNA dynamics
Deterministic two-population model without selection.
In the simplest representation of the model, we discriminated cells that did or did not carry copies of ecDNA. We denoted cells with copies of ecDNA as N + ( t ) and cells without copies of ecDNA with N − ( t ). We can write for the change of these cells in time t :
where \(\upsilon \left( {N^ + \left( t \right)} \right)\) corresponds to the loss rate of random complete asymmetric ecDNA segregation. We found for the fraction of cells carrying ecDNA f + ( t ) in an exponentially growing population:
The fraction of cells carrying ecDNA decreases with approximately 1/ t if ecDNA is neutral. Thus, copies of neutral ecDNA are only present in a small subpopulation of tumor cells.
Deterministic two-population model with selection
The above equations can be modified to allow for a fitness advantage s > 1 for cells carrying ecDNA:
The solution to this set of equations is:
In the case of positive selection, the fraction of cells with ecDNA is \(f^ + \to 1\) . For a sufficiently long time, the tumor will be dominated by cells carrying ecDNA.
Stochastic dynamics of neutral ecDNA
We were also interested in the stochastic properties of ecDNA dynamics in a growing population. Therefore, we moved to a more fine-grained picture and considered the number of cells N k ( t ) with k copies of ecDNA at time t . The dynamic equation for neutral copies of ecDNA becomes:
It is more convenient to work with the cell density ρ instead of cell number N . Normalizing the above equation, we get for the density ρ k of cells with k ecDNA copies:
Moment dynamics for neutral ecDNA copies
With the above equation for the density of cells with k ecDNA copies, we can calculate the moments of the underlying probability density function. In general, the l th moment can by calculated via:
It can be shown that all moments scale with \(M^{\left( l \right)}(t)\approx t^{l - 1}\) and we found explicitly for the first two moments:
\(M^{(1)} = 1\,{{{\mathrm{and}}}}\,M^{\left( 2 \right)}\left( t \right) = t.\)
The mean ecDNA copy number in an exponentially growing population is constant for neutral ecDNA copies. The variance of the ecDNA copy number increases linearly in time.
Stochastic dynamics of ecDNA under positive selection
The above equations can be generalized to accommodate positive selection ( s > 1) for ecDNA copies. The set of dynamic equations for cell densities becomes:
A general solution to these equations is challenging. Nonetheless, important quantities, for example, the moment dynamics and scaling behavior can be calculated explicitly.
Moment dynamics for ecDNA under positive selection
A generalized equation for the dynamics of moments directly follows from the above equations. We have:
This implies for the first moment \(\frac{{\partial M^{\left( 1 \right)}(t)}}{{\partial t}} = (s - 1)\rho _0M^{\left( 1 \right)}(t)\) , which then can be solved for the first moment:
Similarly, the dynamic equation for the second moment becomes \(\frac{{\partial M^{\left( 2 \right)}(t)}}{{\partial t}} = M^{\left( 1 \right)}\left( t \right) + (s - 1)\rho _0M^{\left( 2 \right)}(t)\) and we find
Initially, the first moment increases exponentially. However, with increasing mean copy number, the rate of cells transitioning into a state without ecDNA is decreasing and the increase of the mean ecDNA copy number slowly levels off. Note, for s = 1 we recovered the previous results for the moments of neutral ecDNA amplifications.
Genome editing using CRISPR–Cas9 ribonucleoprotein
Genome editing in COLO320-DM cells was performed using Alt-R S.p. Cas9 Nuclease V3 (catalog no. 1081058; IDT) complexed with sgRNA (Synthego) according to the Synthego RNP transfection protocol using the Neon Transfection System (catalog no. MPK5000; Thermo Fisher Scientific). Briefly, 10 pmol Cas9 protein and 60 pmol sgRNA for each 10 μl reaction were incubated in Neon Buffer R for 10 min at room temperature. Cells were washed with 1× PBS, resuspended in Buffer R and 200,000 cells were mixed with, for the preincubated RNP complex, for each 10-μl reaction. The cell mixture was electroporated according to the manufacturer’s protocol using the following settings: 1,700 V, 20 ms, 1 pulse. Cells were cultured for 10 d afterwards; cell counts and ecDNA copy number data were collected at days 3, 6 and 10. To estimate the ecDNA copy numbers, we performed metaphase chromosome spreading followed by FISH as described above. All sgRNA sequences are in Supplementary Table 3 .
FISH probes
The following probes were used for FISH as indicated: Zyto Light SPEC CDK4/CEN 12 Dual Color Probe (ZytoVision); Zyto Light SPEC MYCN/2q11 Dual Color Probe (ZytoVision); Empire Genomics EGFR FISH Probe; Empire Genomics MYC FISH Probe; Empire Genomics FGFR2 FISH Probe; Empire Genomics CDK4 FISH Probe; Empire Genomics MYCN FISH Probe.
The following antibodies were used at concentrations of 1:100–1:200 for immunofluorescence and 1:1,000 for immunoblotting (unless otherwise indicated in specific Methods sections): Aurora B Polyclonal Antibody (catalog no. A300-431A; Thermo Fisher Scientific); EGFRvIII monoclonal antibody 806 (ref. 36 ); anti-mouse Alexa Fluor 488.
Statistics and reproducibility
Sample sizes for the biological experiments analyzing copy number distributions were informed by stochastic simulations. Investigators were not blinded to experimental groups.
Reporting summary
Further information on research design is available in the Nature Research Reporting Summary linked to this article.
Data availability
This study did not generate any new nucleic acid sequencing data. All data and materials, including cell constructs, will be made available upon reasonable request from the corresponding author. Source data are provided with this paper.
Code availability
Code for the stochastic simulations of random ecDNA segregations are available at GitHub-BenWernerScripts/ECDNA-DYNAMICS.
Merlo, L. M. F., Pepper, J. W., Reid, B. J. & Maley, C. C. Cancer as an evolutionary and ecological process. Nat. Rev. Cancer 6 , 924–935 (2006).
Article CAS Google Scholar
Greaves, M. & Maley, C. C. Clonal evolution in cancer. Nature 481 , 306–313 (2012).
McGranahan, N. & Swanton, C. Clonal heterogeneity and tumor evolution: past, present, and the future. Cell 168 , 613–628 (2017).
McGranahan, N. & Swanton, C. Biological and therapeutic impact of intratumor heterogeneity in cancer evolution. Cancer Cell 27 , 15–26 (2015).
Abbosh, C. et al. Phylogenetic ctDNA analysis depicts early-stage lung cancer evolution. Nature 545 , 446–451 (2017).
Barthel, F. P. et al. Longitudinal molecular trajectories of diffuse glioma in adults. Nature 576 , 112–120 (2019).
Jamal-Hanjani, M. et al. Tracking the evolution of non-small-cell lung cancer. N. Engl. J. Med. 376 , 2109–2121 (2017).
Burrell, R. A., McGranahan, N., Bartek, J. & Swanton, C. The causes and consequences of genetic heterogeneity in cancer evolution. Nature 501 , 338–345 (2013).
Nathanson, D. A. et al. Targeted therapy resistance mediated by dynamic regulation of extrachromosomal mutant EGFR DNA. Science 343 , 72–76 (2014).
Furnari, F. B., Cloughesy, T. F., Cavenee, W. K. & Mischel, P. S. Heterogeneity of epidermal growth factor receptor signalling networks in glioblastoma. Nat. Rev. Cancer 15 , 302–310 (2015).
Watkins, T. B. K. et al. Pervasive chromosomal instability and karyotype order in tumour evolution. Nature 587 , 126–132 (2020).
Kim, C. et al. Chemoresistance evolution in triple-negative breast cancer delineated by single-cell sequencing. Cell 173 , 879–893.e13 (2018).
Vasan, N., Baselga, J. & Hyman, D. M. A view on drug resistance in cancer. Nature 575 , 299–309 (2019).
Kim, H. et al. Extrachromosomal DNA is associated with oncogene amplification and poor outcome across multiple cancers. Nat. Genet. 52 , 891–897 (2020).
Turner, K. M. et al. Extrachromosomal oncogene amplification drives tumour evolution and genetic heterogeneity. Nature 543 , 122–125 (2017).
Lundberg, G. et al. Binomial mitotic segregation of MYCN -carrying double minutes in neuroblastoma illustrates the role of randomness in oncogene amplification. PLoS ONE 3 , e3099 (2008).
Article Google Scholar
Shoshani, O. et al. Chromothripsis drives the evolution of gene amplification in cancer. Nature 591 , 137–141 (2021).
Yi, E. et al. Live-cell imaging shows uneven segregation of extrachromosomal DNA elements and transcriptionally active extrachromosomal DNA hubs in cancer. Cancer Discov. 12 , 468–483 (2022).
Thompson, S. L. & Compton, D. A. Chromosome missegregation in human cells arises through specific types of kinetochore-microtubule attachment errors. Proc. Natl Acad. Sci. USA 108 , 17974–17978 (2011).
Fuller, B. G. et al. Midzone activation of aurora B in anaphase produces an intracellular phosphorylation gradient. Nature 453 , 1132–1136 (2008).
Tasan, I. et al. CRISPR/Cas9-mediated knock-in of an optimized TetO repeat for live cell imaging of endogenous loci. Nucleic Acids Res. 46 , e100 (2018).
Grimm, J. B. et al. A general method to optimize and functionalize red-shifted rhodamine dyes. Nat. Methods 17 , 815–821 (2020).
Gerlinger, M. et al. Intratumor heterogeneity and branched evolution revealed by multiregion sequencing. N. Engl. J. Med. 366 , 883–892 (2012).
Qazi, M. A. et al. Intratumoral heterogeneity: pathways to treatment resistance and relapse in human glioblastoma. Ann. Oncol. 28 , 1448–1456 (2017).
Gillespie, D. T. General method for numerically simulating stochastic time evolution of coupled chemical-reactions. J. Comput. Phys. 22 , 403–434 (1976).
Møller, H. D. et al. CRISPR-C: circularization of genes and chromosome by CRISPR in human cells. Nucleic Acids Res. 46 , e131 (2018).
PubMed PubMed Central Google Scholar
Kaufman, R. J., Brown, P. C. & Schimke, R. T. Amplified dihydrofolate reductase genes in unstably methotrexate-resistant cells are associated with double minute chromosomes. Proc. Natl Acad. Sci. USA 76 , 5669–5673 (1979).
Wu, S. et al. Circular ecDNA promotes accessible chromatin and high oncogene expression. Nature 575 , 699–703 (2019).
Paffhausen, T., Schwab, M. & Westermann, F. Targeted MYCN expression affects cytotoxic potential of chemotherapeutic drugs in neuroblastoma cells. Cancer Lett. 250 , 17–24 (2007).
Cen, L. et al. p16-Cdk4-Rb axis controls sensitivity to a cyclin-dependent kinase inhibitor PD0332991 in glioblastoma xenograft cells. Neuro Oncol. 14 , 870–881 (2012).
Hung, K. L. et al. ecDNA hubs drive cooperative intermolecular oncogene expression. Nature 600 , 731–736 (2021).
Ambros, P. F. et al. International consensus for neuroblastoma molecular diagnostics: report from the International Neuroblastoma Risk Group (INRG) Biology Committee. Br. J. Cancer 100 , 1471–1482 (2009).
Naito, Y., Hino, K., Bono, H. & Ui-Tei, K. CRISPRdirect: software for designing CRISPR/Cas guide RNA with reduced off-target sites. Bioinformatics 31 , 1120–1123 (2015).
Xie, L. et al. 3D ATAC-PALM: super-resolution imaging of the accessible genome. Nat. Methods 17 , 430–436 (2020).
Guzmán, C., Bagga, M., Kaur, A., Westermarck, J. & Abankwa, D. ColonyArea: an ImageJ plugin to automatically quantify colony formation in clonogenic assays. PLoS ONE 9 , e92444 (2014).
Jungbluth, A. A. et al. A monoclonal antibody recognizing human cancers with amplification/overexpression of the human epidermal growth factor receptor. Proc. Natl Acad. Sci. USA 100 , 639–644 (2003).
Download references
Acknowledgements
The eDyNAmiC research was facilitated by Cancer Grand Challenges (grant no. CGCSDF-2021\100007) with support from Cancer Research UK and the National Cancer Institute. This study was supported by a grant from the National Brain Tumour Society and National Institutes of Health (NIH) grant no. R01-CA238349 to P.S.M. H.Y.C. is an investigator of the Howard Hughes Medical Institute and was also supported by NIH grant no. R35-CA209919. A Barts Charity Lectureship MGU045 and UKRI Future Leaders Fellowship supported B.W. The UCSD microscopy core is supported by National Institute of Neurological Disorders and Stroke (no. NS047101). NIH grant nos. U24CA264379 and 1R01GM114362 supported V.B. The A.P. Giannini Foundation supported J.C.R. The German Research Foundation (grant no. 398299703) supported A.G.H. This project has received funding from the European Research Council (ERC) under the European Union’s Horizon 2020 research and innovation programme (grant no. 949172). The Cancer Prevention and Research Institute of Texas (no. RR210034) supported S.W. We thank C. Zhang from the Howard Chang Laboratory for providing technical assistance with the CRISPR-C experiments. C.S. is a Royal Society Napier Research Professor (no. RSRP\R\210001). This work was supported by the Francis Crick Institute, which receives its core funding from Cancer Research UK (no. FC001169), the UK Medical Research Council (no. FC001169) and the Wellcome Trust (no. FC001169). This research was funded in whole, or in part, by the Wellcome Trust (no. FC001169). For the purpose of open access, the authors applied a CC BY public copyright licence to any author-accepted manuscript version arising from this submission. C.S. is funded by Cancer Research UK (TRACERx (no. C11496/A17786), PEACE (C416/A21999) and CRUK Cancer Immunotherapy Catalyst Network), Cancer Research UK Lung Cancer Centre of Excellence (no. C11496/A30025), the Rosetrees Trust, Butterfield and Stoneygate Trusts, NovoNordisk Foundation (ID16584), Royal Society Professorship Enhancement Award (no. RP/EA/180007), the National Institute for Health Research Biomedical Research Centre at University College London Hospitals, the Cancer Research UK-University College London Centre, Experimental Cancer Medicine Centre and the Breast Cancer Research Foundation (no. BCRF 20-157). This work was supported by a Stand Up To Cancer (SU2C)‐LUNGevity-American Lung Association Lung Cancer Interception Dream Team Translational Research Grant (grant no. SU2C-AACR-DT23-17 to S. M. Dubinett and A. E. Spira). SU2C is a division of the Entertainment Industry Foundation. Research grants are administered by the American Association for Cancer Research, the Scientific Partner of SU2C. C.S. is in receipt of an ERC Advanced Grant (PROTEUS) from the European Research Council under the European Union’s Horizon 2020 research and innovation programme (grant no. 835297). Funding from the Vienna Science and Technology Fund (no. LS18-111), Austrian Science Fund (nos. I4162 and P35841-B) and St. Anna Kinderkrebsforschung supported S.T.-M. We thank D. Nathanson for provision of clinical data. We thank A. Desai for usage of equipment and experimental advice.
Author information
These authors contributed equally: Joshua T. Lange, John C. Rose, Celine Y. Chen, Yuriy Pichugin.
These authors jointly supervised this work: Weini Huang, Howard Y. Chang, Vineet Bafna, Anton G. Henssen, Benjamin Werner, Paul S. Mischel.
Authors and Affiliations
Department of Pathology, Stanford University School of Medicine, Stanford, CA, USA
Joshua T. Lange, Jun Tang & Paul S. Mischel
ChEM-H, Stanford University, Stanford, CA, USA
Center for Personal Dynamic Regulomes, Stanford University School of Medicine, Stanford, CA, USA
John C. Rose, King L. Hung, Kathryn E. Yost, Quanming Shi & Howard Y. Chang
Department of Pediatric Oncology/Hematology, Charité-Universitätsmedizin Berlin, Berlin, Germany
Celine Y. Chen & Anton G. Henssen
Department of Evolutionary Theory, Max Planck Institute for Evolutionary Biology, Plön, Germany
Yuriy Pichugin
Department of Ecology and Evolutionary Biology, Princeton University, Princeton, NJ, USA
Janelia Research Campus, Howard Hughes Medical Institute, Ashburn, VA, USA
Liangqi Xie & Zhe Liu
Department of Molecular and Cell Biology, Li Ka Shing Center for Biomedical and Health Sciences, California Institute for Regenerative Medicine Center of Excellence, University of California, Berkeley, CA, USA
Liangqi Xie
University of California San Diego Light Microscopy Core Facility, Department of Neurosciences, University of California San Diego, La Jolla, CA, USA
Marcella L. Erb
Department of Computer Science and Engineering, University of California San Diego, La Jolla, CA, USA
Utkrisht Rajkumar & Vineet Bafna
Children’s Medical Center Research Institute, University of Texas Southwestern Medical Center, Dallas, TX, USA
St. Anna Children’s Cancer Research Institute, Vienna, Austria
Sabine Taschner-Mandl & Marie Bernkopf
Cancer Evolution and Genome Instability Laboratory, The Francis Crick Institute, London, UK
Charles Swanton
Cancer Research UK Lung Cancer Centre of Excellence, University College London Cancer Institute, London, UK
Department of Medical Oncology, University College London Hospitals, London, UK
Group of Theoretical Biology, The State Key Laboratory of Biocontrol, School of Life Science, Sun Yat-sen University, Guangzhou, China
Weini Huang
Department of Mathematics, Queen Mary University of London, London, UK
Howard Hughes Medical Institute, Stanford University, Stanford, CA, USA
Howard Y. Chang
Experimental and Clinical Research Center, Max Delbrück Center for Molecular Medicine and Charité-Universitätsmedizin Berlin, Berlin, Germany
Anton G. Henssen
German Cancer Consortium and German Cancer Research Center, Heidelberg, Germany
Berlin Institute of Health, Berlin, Germany
Evolutionary Dynamics Group, Centre for Cancer Genomics and Computational Biology, Barts Cancer Institute, Queen Mary University of London, London, UK
Benjamin Werner
You can also search for this author in PubMed Google Scholar
Contributions
J.T.L., V.B., B.W. and P.S.M. conceived the project. J.T.L., J.C.R., C.Y.C., L.X., J.T., K.L.H., K.E.Y., Q.S., M.L.E., S.T. and M.B. performed the experiments. Y.P., W.H., V.B. and B.W. performed the computational modeling. J.T.L., J.C.R., C.Y.C., Y.P., L.X., J.T., K.L.H., K.E.Y., Q.S. and U.R. analyzed the data, guided by S.W., C.S., Z.L., W.H., H.Y.C., V.B., A.G.H., B.W. and P.S.M. J.T.L., W.H., V.B., B.W. and P.S.M. wrote the manuscript with feedback from all authors.
Corresponding authors
Correspondence to Weini Huang , Howard Y. Chang , Vineet Bafna or Paul S. Mischel .
Ethics declarations
Competing interests.
P.S.M. is cofounder of Boundless Bio. He has equity and chairs the scientific advisory board, for which he is compensated. V.B. is a cofounder, consultant, scientific advisory board member and has an equity interest in Boundless Bio. and Abterra Biosciences. The terms of this arrangement have been reviewed and approved in accordance with its conflict-of-interest policies. H.Y.C. is a cofounder of Accent Therapeutics, Boundless Bio, Cartography Biosciences, Orbital Therapeutics, and advisor of 10X Genomics, Arsenal Biosciences and Spring Discovery. J.T.L. was employed by Boundless Bio after completing this work. C.S. acknowledges grant support from AstraZeneca, Boehringer-Ingelheim, Bristol Myers Squibb, Pfizer, Roche-Ventana, Invitae (previously ArcherDX, collaboration in minimal residual disease sequencing technologies) and Ono Pharmaceutical. He is an AstraZeneca advisory board member and chief investigator for the AZ MeRmaiD 1 and 2 clinical trials and is also chief investigator of the NHS-Galleri trial. He has consulted for Amgen, AstraZeneca, Pfizer, Novartis, GSK, MSD, Bristol Myers Squibb, Illumina, Genentech, Roche-Ventana, GRAIL, Medicxi, Metabomed, Bicycle Therapeutics, Roche Innovation Centre Shanghai and the Sarah Cannon Research Institute. C.S. had stock options in ApoGen Biotechnologies and GRAIL until June 2021, has currently stock options in Epic Bioscience and Bicycle Therapeutics, and has stock options and is a cofounder of Achilles Therapeutics. C.S. holds patents relating to assay technology to detect tumor recurrence (PCT/GB2017/053289), targeting neoantigens (PCT/EP2016/059401), identifying patent response to immune checkpoint blockade (PCT/EP2016/071471), determining human leukocyte antigen loss of heterozygosity (PCT/GB2018/052004), predicting survival rates of patients with cancer (PCT/GB2020/050221), identifying patients who respond to cancer treatment (PCT/GB2018/051912), a US patent relating to detecting tumor mutations (PCT/US2017/28013), methods for lung cancer detection (US20190106751A1) and both European and US patents related to identifying insertion/deletion mutation targets (PCT/GB2018/051892). The other authors declare no competing interests.
Peer review
Peer review information.
Nature Genetics thanks Andrew Futreal and the other, anonymous, reviewer(s) for their contribution to the peer review of this work.
Additional information
Publisher’s note Springer Nature remains neutral with regard to jurisdictional claims in published maps and institutional affiliations.
Extended data
Extended data fig. 1 overview of strategies to develop and test rules of ecdna behavior with mathematical modeling, computer simulations, cell line and patient data..
a, Overview of the strategies employed to develop and test experimental, patient and theoretical behavior of ecDNA.
Extended Data Fig. 2 ecDNA segregation in cancer cell lines across cancer type and amplified oncogene.
a, Representative metaphase FISH images for cell lines used to quantify segregation dynamics in Fig. 1 Scale bars 10μm. b, The same daughter cells analyzed in Fig. 1c were analyzed by quantifying the pixel intensity of FISH signal in each daughter cell, as a proxy for ecDNA number. Agreement between theoretical predictions (dashed lines) and observation (histograms) shown by KS tests. c, Quantile-quantile plots comparing the distribution of measured ecDNA segregation fraction and simulated random ecDNA segregation.
Extended Data Fig. 3 Live cell tracking of ecDNA through insertion of Tet-O array into the ecDNA of PC3 cells.
a, Representative images of PC3 parental and PC3-TetO cell lines showing extensive MYC amplification on both. PC3-TetO shows significant TetO FISH signal on multiple ecDNA bodies as well. b, PCR amplification of 96-mer TetO repeats. c, PCR amplification of 96-mer TetO repeats from DNA isolated from PC3-TetO cells confirming insertion. d, Sanger sequencing of PCR amplification product from PC3-TetO cells. Both left and right junctions were repaired by homologous recombination at the insertion site.
Extended Data Fig. 4 ecDNA copy number distribution in cell lines.
a, Quantile-quantile plots comparing ecDNA copy number distributions in cell line populations with simulated populations following random segregation of ecDNA.
Extended Data Fig. 5 ecDNA heterogeneity in patient tumours.
a, Histograms of ecDNA copy number assessed by interphase FISH on patient tumor tissue from neuroblastoma (NB) patients with MYCN amplification. b, Quantile-quantile plots for ecDNA copy number comparing measured ecDNA number from patient tissue FISH and simulated ecDNA number from simulated tumour growth.
Extended Data Fig. 6 ecDNA dynamically responds to therapeutics.
a, Schematic depicting the trends in ecDNA copy number frequency and copy number under neutral and positive selection. b, Simulations showing ecDNA prevalence in populations derived from a single ecDNA+ cell with ecDNA under positive or neutral selection. Positive selection s = 3; neutral s = 1. c, Quantification of ecDNA frequency in patient and cell line samples grouped by amplified oncogene compared to expected levels given varying levels of selection as indicated. d, Quantification of ecDNA numbers at Day 6 and Day 10 after CRISPR cutting of regions of the COLO320-DM genome, either on or off of ecDNA. Shows clear evidence for selection of ecDNA both by the severe drop in copy number when targeted and the inidcation that the copy number begins to return to initial levels. Note ecDNA_MYC at day 6 is severely limited in its growth and only 6 metaphases were able to be identified and imaged. P-values shown from 2 sided t-tests.
Extended Data Fig. 7 ecDNA dynamically responds to therapeutics.
a, Representative images of metaphase spread FISH from isogenic GBM39 cell line. b, Stochastic simulations of Shannon diversity under either either random ecDNA inheritance (GBM39-EC) or canonical chromosomal inheritance (GBM39-HSR). 100 stochastic simulations were run for each condition. Boxplots are shown with line at median and box ranging from 25th to 75th percentile, whiskers extendting to most extreme value. c, Flow cytometry analysis of EGFR protein expression in isogenic GBM39-EC and GBM39-HSR cell lines shows pattern of heterogeneity similar to that seen in copy number. X-CV quantifies the % coefficient of variation for the two samples. We used FSC-A/SSC-A to locate the major cell population, and FSC-H/FSC-W to gate the single cells. We used a negative control sample (secondary only) to adjust the voltage for the Alexa-Fluor488 channel. d, Representative images of TR14 cells treated with Abemaciclib or Palbociclib for 60 days. CDK4 FISH signal shown in green, CEN12 control FISH probe shown in red. e, Quantification of experiment described in d shows significant shift in CDK4 ecDNA copy number distribution under both drug conditions. f, Quantification of EGFR ecDNA in GBM39-EC cells after short-term treatment with erlotinib shows rapid change in ecDNA copy number distribution. Lines indicate medians. P values calculated using Mann-Whitney tests. * p ≤ 0.05; **** p ≤ 0.0001. Scale bars 10 µm.
Extended Data Fig. 8 ecDNA dynamics correlate with formation of resistance.
a, Treatment of long term palbociclib resistant populations of TR14 cells with palbociclib or abemaciclib, showing resistance to treatment. b, Treatment of long term abemaciclib resistant populations of TR14 cells with palbociclib or abemaciclib showing resistance to treatment. c, Validation of increased ecDNA copy number by qPCR for CDK4. (a-c) Data presented as mean +/− standard deviation from 3 technical replicates. d, Crystal violet staining of TR14 cells re-challenged with palbociclib or abemaciclib after development of resistance, or not (DMSO). e, Quantification of d showing resistance in populations treated with CDK4 inhibitors for 60 days. Data presented as mean +/− standard deviation from 3 technical replicates.
Supplementary information
Supplementary information.
Supplementary Figs. 1–14.
Reporting Summary
Supplementary video 1.
Time-lapse video showing unequal segregation of ecDNA.
Supplementary Table 3
PCR and CRISPR oligonucleotide sequences
Source Data Fig. 1
Unprocessed gel.
Source Data Fig. 2
Rights and permissions.
Open Access This article is licensed under a Creative Commons Attribution 4.0 International License, which permits use, sharing, adaptation, distribution and reproduction in any medium or format, as long as you give appropriate credit to the original author(s) and the source, provide a link to the Creative Commons license, and indicate if changes were made. The images or other third party material in this article are included in the article’s Creative Commons license, unless indicated otherwise in a credit line to the material. If material is not included in the article’s Creative Commons license and your intended use is not permitted by statutory regulation or exceeds the permitted use, you will need to obtain permission directly from the copyright holder. To view a copy of this license, visit http://creativecommons.org/licenses/by/4.0/ .
Reprints and permissions
About this article
Cite this article.
Lange, J.T., Rose, J.C., Chen, C.Y. et al. The evolutionary dynamics of extrachromosomal DNA in human cancers. Nat Genet 54 , 1527–1533 (2022). https://doi.org/10.1038/s41588-022-01177-x
Download citation
Received : 16 February 2022
Accepted : 01 August 2022
Published : 19 September 2022
Issue Date : October 2022
DOI : https://doi.org/10.1038/s41588-022-01177-x
Share this article
Anyone you share the following link with will be able to read this content:
Sorry, a shareable link is not currently available for this article.
Provided by the Springer Nature SharedIt content-sharing initiative
This article is cited by
Machine learning-based extrachromosomal dna identification in large-scale cohorts reveals its clinical implications in cancer.
- Shixiang Wang
Nature Communications (2024)
scCircle-seq unveils the diversity and complexity of extrachromosomal circular DNAs in single cells
- Jinxin Phaedo Chen
- Constantin Diekmann
- Nicola Crosetto
Extrachromosomal DNA in cancer
- Xiaowei Yan
- Paul Mischel
- Howard Chang
Nature Reviews Cancer (2024)
Etiology of super-enhancer reprogramming and activation in cancer
- Royce W. Zhou
- Ramon E. Parsons
Epigenetics & Chromatin (2023)
Disentangling extrachromosomal circular DNA heterogeneity in single cells with scEC&T-seq
Nature Genetics (2023)
Quick links
- Explore articles by subject
- Guide to authors
- Editorial policies
Sign up for the Nature Briefing: Cancer newsletter — what matters in cancer research, free to your inbox weekly.


An official website of the United States government
The .gov means it’s official. Federal government websites often end in .gov or .mil. Before sharing sensitive information, make sure you’re on a federal government site.
The site is secure. The https:// ensures that you are connecting to the official website and that any information you provide is encrypted and transmitted securely.
- Publications
- Account settings
Preview improvements coming to the PMC website in October 2024. Learn More or Try it out now .
- Advanced Search
- Journal List
- Heredity (Edinb)
- v.129(1); 2022 Jul
Inheritance through the cytoplasm
M. florencia camus.
1 Department of Genetics, Evolution and Environment, University College London, London, UK
Bridie Alexander-Lawrie
2 Biology Department, New Mexico Institute of Mining and Technology, Socorro, NM USA
Joel Sharbrough
Gregory d. d. hurst.
3 Institute of Infection, Veterinary and Ecological Sciences, University of Liverpool, Liverpool, England
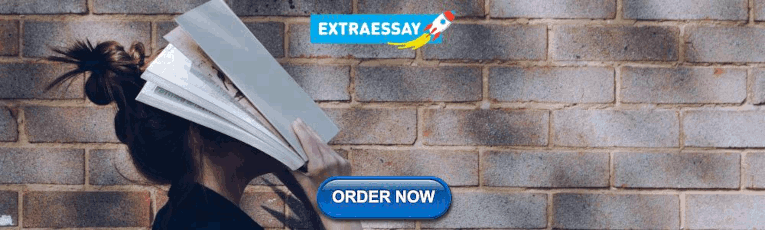
Associated Data
Most heritable information in eukaryotic cells is encoded in the nuclear genome, with inheritance patterns following classic Mendelian segregation. Genomes residing in the cytoplasm, however, prove to be a peculiar exception to this rule. Cytoplasmic genetic elements are generally maternally inherited, although there are several exceptions where these are paternally, biparentally or doubly-uniparentally inherited. In this review, we examine the diversity and peculiarities of cytoplasmically inherited genomes, and the broad evolutionary consequences that non-Mendelian inheritance brings. We first explore the origins of vertical transmission and uniparental inheritance, before detailing the vast diversity of cytoplasmic inheritance systems across Eukaryota. We then describe the evolution of genomic organisation across lineages, how this process has been shaped by interactions with the nuclear genome and population genetics dynamics. Finally, we discuss how both nuclear and cytoplasmic genomes have evolved to co-inhabit the same host cell via one of the longest symbiotic processes, and all the opportunities for intergenomic conflict that arise due to divergence in inheritance patterns. In sum, we cannot understand the evolution of eukaryotes without understanding hereditary symbiosis.
Introduction
The vast majority of genes in eukaryotes are located within chromosomal structures in the nucleus of the cell. These transmit copies of themselves to the next generation via meiosis involving strict segregation. However, eukaryotic cells also harbour smaller genomes, which reside in the cytoplasm, including: mitochondrial DNA, chloroplast DNA, and symbiont genomes. Interestingly, cytoplasmic genetic elements have been shown to have very different inheritance patterns to classic Mendelian nuclear chromosomes. The first documented evidence for this came from Carl Correns research on the four o’clock plant Mirabilis jalapa , in which he detailed the non-Mendelian inheritance of leaf colour (Correns 1909 ). Inheritance, in this case, was strictly maternal: a seed derived from an ovule from a non-green stem gave rise to non-green progeny, irrespective of the source of pollen. By 1952, the evidence of various forms of cytoplasmically inherited elements (CIEs) had grown, leading Joshua Lederberg to synthesise the inheritance of cellular organelles and symbionts into one framework in his treatise “Cell genetics and hereditary symbiosis” (Lederberg 1952 ). Furthermore, evidence for diversity in inheritance patterns (paternal or biparental) of CIEs started accumulating for a wide range of taxa (Birky 2001 ).
Since then, studies have demonstrated that CIEs are diverse and important - in many cases, encoding key aspects of organismal function. Cytoplasmically inherited elements vary in their level of integration with the host - in the case of organelles, the proteome is jointly encoded in nuclear and organellar DNA, in addition to integration into cellular physiology. For obligate microbial symbionts, anatomical and physiological integration are evident but generally without trafficking of host proteins into the microbe; they are commonly present in particular tissues and have host organised vertical transmission. Other microbial symbionts are less integrated, present more diffusely in the host and invade the germ line to gain vertical transmission.
In this review, we describe the diversity of inheritance systems of CIEs, and highlight the evolutionary consequences that these inheritance systems bring to cellular, organismal and population dynamics (Fig. (Fig.1). 1 ). For this, we focus on the three main groups of CIEs: mitochondrial DNA, chloroplast DNA, and symbiont genomes. We begin by outlining the origins of cytoplasmic inheritance and the evolution of uniparental inheritance, documenting the diversity of cytoplasmic inheritance systems so far observed. We discuss the diversity and patterns of genome organisation for cytoplasmic elements and examine the population genetics of CIEs, highlighting the tension between within- and between-individual spread. We summarise the evidence for the adaptive importance of cytoplasmic genes before detailing coadaptation between the cytoplasm and the nucleus, and amongst cytoplasmic components.

New mutations that arise immediately produce intra-organellar variation depicted here by differently coloured nucleoids (Mitochondria & Chloroplast panel). If mutations (differently coloured nucleoids) spread between organelles, variation between organelles is observed (Plant Cell panel). Note that mitochondria often form reticulated syncytia, rather than discrete compartments, in contrast to chloroplasts, which may facilitate recombination and therefore spread of mutations throughout the cell. Intracellular variation can give rise to intra-tissue variation, depicted here in the form of a variegated leaf ( Psychotria leaf panel). Psychotria also features bacterial leaf nodules (dark green circles) that contain Burkholderia bacteria which are vertically inherited through the seed. Variation within tissues can then give rise to variation across tissues ( Psychotria Plant panel). If germlines are segregated late, this can result in distinct alleles being propagated to the next generation from different parts of the plant. As a consequence, the variation that originated at the individual organelle level can finally be observed between individuals within populations ( Psychotria population panel).
The evolutionary origins of vertical transmission and uniparental inheritance
The ancestors of current cytoplasmically inherited genetic material were free-living organisms (Sagan 1967 ), but how cytoplasmic inheritance originated and came to be limited to one sex remains an open question. For microeukaryotes (and indeed for the ancestral protoeukaryote) the presence of a microbe inside the cytoplasm would de facto produce inheritance on cell division. This would form a continuous system if replication of the microbe within the cell was occurring. Thus, the primary drive to cytoplasmic inheritance is intracellular location and replication, which could be initially host driven (symbiont capture) or symbiont driven (infection of the host, or escape from a phagolysosome).
Notably, the rate of vertical transmission for symbionts that can also transmit infectiously is evolvable: symbionts with mixed modes of transmission that are kept in continuously growing host populations (where cell division provides ample opportunity for vertical transmission) evolve a stronger tendency for heritable transmission compared to those kept in populations at carrying capacity (where the opportunity for vertical transmission is limited) (Magalon et al. 2010 ). Thus, there is a trajectory in which infection through the environment is lost.
When vertical transmission does evolve, there are two primary consequences. First, the population size of symbionts and mixing of strains declines, reducing within host conflicts. Second, symbiont fitness becomes a product not only of host survival but additionally host reproduction. Both of these processes drive the symbiosis towards the mutualism end of the mutualism-parasitism continuum, with current models indicating restrictions on symbiont diversity through bottlenecks and reduced mixing opportunities being most important in this transition, through quelling the conflicts associated with within-host competition (Leeks et al. 2019 ). Later transitions would then involve adaptation to the intracellular environment with correlated loss of capacity for free-living life and infection processes. All of these are reflected in the reductive genome evolution pattern commonly observed in heritable symbionts (Moran et al. 2008 ).
The evolution of inheritance for symbionts of multicellular hosts also has its origins in the association of free-living organisms, with a transition from symbiosis where the parties reform symbiosis through environmental association each generation to vertical transmission. Indeed, some symbiont clades include both symbionts acquired through the environment and heritable symbionts (e.g., (Drew et al. 2021 )). Vertical transmission may arise passively through spatial structure (symbionts from a parent are more likely to infect progeny of that parent through proximity), actively through selection on the host to ensure passage of a beneficial symbiont (host driven vertical transmission) or actively through selection on the microbe to infect the next generation through the germ line. Evolution towards vertical transmission may be constrained through location (e.g., soil microbe-plant root associations have no proximity to the germ line), and made less likely in environments where partner availability is high (e.g., aquatic environments (Douglas 1998 )) or where the symbiont has utility only in a restricted part of host life history (Hartmann et al. 2017 ).
Most cytoplasmic genetic material is inherited uniparentally - that is to say from one parent only. It is notable that uniparental inheritance is not restricted to anisogamous species - it is also commonly observed in isogamous microeukaryotes associated with mating types. This observation implies that uniparental inheritance is not a simple by-product of gamete size, but rather is an evolved state (Hurst and Hamilton 1992 ). Anisogamy itself is considered by some as a potential onward adaptive mechanism for imposing uniparental inheritance, in contrast to the passive view that uniparental inheritance is a by-product of anisogamy (Hurst 1990 ).
Evolutionary drivers of uniparental inheritance include the benefit of preventing conflicts and the damage from cytoplasmic mixing. Under a biparental inheritance scenario, we would expect a heteroplasmic state, in which multiple distinct forms of the CIE exist within the same cell, to be the norm. For mitochondria, heteroplasmy interferes with cell functioning, with empirical work demonstrating that it can cause organismal dysfunction (Nissanka and Moraes 2020 ). Moreover, heteroplasmy reduces the variance between cells, and if this is happening in the germ line, it then reduces the efficacy of selection (Radzvilavicius et al. 2016 ). Mathematical models show that selection against heteroplasmy can lead to the fixation of uniparental inheritance in an ancestrally biparental population (Christie et al. 2015 ; Christie and Beekman 2017 ). Mathematical models indicate that mitonuclear coadaptation is improved with uniparental inheritance and mitochondrial bottlenecks under a wide range of conditions (Hadjivasiliou et al. 2012 ).
Beyond simple maternal inheritance
Whilst the majority of anisogamous species transmit CIEs uniparentally, via the egg (Birky 2001 ), heteroplasmy via paternal leakage can occur when maternal inheritance is not strictly enforced (see Table Table1 1 and Supplementary Table S1 for examples). Most species appear to exhibit some degree of leakage if sampled carefully enough (e.g., (Wagner et al. 1991 ; Kvist et al. 2003 ; Fontaine et al. 2007 ; Bentley et al. 2010 ; Nunes et al. 2013 )), but the processes that contribute to variation in leakage rates are not well understood. By contrast, a variety of mechanisms that reinforce maternal inheritance by eliminating and/or silencing paternally derived elements have been documented (Sato and Sato 2013 , 2017 ). Still, paternally derived CIEs occasionally experience positive selection, resulting in introgression and even replacement of the maternal CIE lineage by the paternal CIE lineage (Wolff et al. 2013 ).
Alternative cytoplasmic inheritance mechanisms observed in plant, fungal, and animal mitochondria and chloroplasts.
See Supplementary Table S1 for expanded view.
Notably, there exists a biological distinction between paternal leakage versus paternal inheritance of CIEs. Paternal leakage, in which CIEs are inherited mostly maternally, but with some minor contribution from the paternal gamete, provides at least some opportunity for genetic exchange between CIEs from different lineages. By contrast, CIE lineages from separate parents are not expected to interact or recombine under systems of paternal inheritance, even if only occasional, in which all of the CIEs present in an individual host are paternally derived (e.g., Ross et al. 2016 ). The implications are that paternal leakage allows for breakdown of linkage disequilibrium between separate nucleotides of the CIE genome, such that beneficial mutations can be decoupled from deleterious genomic backgrounds and vice versa (Hill and Robertson 1966 ).
Evolutionary transitions from maternal to paternal inheritance are not especially common, but do happen (Table (Table1, 1 , Supplementary Table S1 ). Interestingly, shifts in one organelle do not always affect the other cytoplasmic elements within the cell (but see Pelargonium (Weihe et al. 2009 ); Sequoia (Neale et al. 1989 )), indicating that the genetic machinery regulating cytoplasmic inheritance is independent across separate CIEs. For example, in Musa acuminata (banana), the mitochondria are inherited paternally and the chloroplasts are inherited maternally (Fauré et al. 1994 ). Some other examples of mixed uniparental inheritance include cucumbers, melons (Havey 1997 ) (in contrast to the rest of the Cucurbitaceae (Havey et al. 1998 )), which follow the same pattern as banana, and loblolly pines, which feature maternally transmitted mitochondria and paternally transmitted chloroplasts (Neale and Sederoff 1989 ).
When both parents contribute CIEs to the offspring, it is probable that cells benefit from biparental mitochondrial inheritance as it provides higher individual genetic diversity, which leads to reduced susceptibility to deleterious mutation. However, this naturally leads to competition and conflict between lineages. Recent work has shown that biparental inheritance has the potential to be beneficial and remain stable for hybridising populations if the fitness cost of mitonuclear incompatibilities in hybrids is greater than a stable state of heteroplasmy (which is also detrimental to the individual, but less so) (Allison et al. 2021 ). In hybridised Pelargonium (geranium) cultivars, for example, mitochondrial and plastid biparental inheritance has been seen alongside chloroplast variegation (Baur 1908 ). In these hybrids, differing ratios of maternally and paternally derived cytoplasmic genomes were observed across different tissues (Weihe et al. 2009 ). Based on the phylogenetic distribution of biparental inheritance systems, it seems that the balance of reduced impact of deleterious mutations vs. increased conflict between CIE lineages favours the latter, as relatively few examples of fully biparentally inherited CIEs exist.
A particularly interesting case of biparental inheritance of mitochondria is that of doubly-uniparental inheritance (DUI) in bivalves, in which the paternal mtDNA (M-type) is passed down to male offspring and maternal mtDNA (F-type) is passed down to offspring of both sexes (Breton et al. 2007 ; Passamonti and Ghiselli 2009 ; Zouros 2013 ). In this scheme, two independently evolving mtDNA lineages are found in male individuals, but the sperm produced only contain the lineage they inherited paternally (Ladoukakis and Zouros 2017 ). The paternal mitochondria are largely confined to the gonad and the maternal mitochondria the soma, resulting in sperm that carry only the male mitochondrial line (Ghiselli et al. 2020 ). The separate localisation of M-type vs. F-type mtDNAs also makes it less likely that recombination between mtDNA lineages occurs (but see (Zouros 2000 ; Burzyński et al. 2003 ; Passamonti et al. 2003 ; Breton et al. 2006 ; Stewart et al. 2009 )).
Heritable microbes are commonly considered as exclusively transmitted from mother to offspring only; however, this rule is not absolute. Many heritable viruses show biparental inheritance, commonly with higher fidelity through the egg than sperm (Roossinck 2010 ). For heritable bacteria, early studies of Volvox carteri revealed efficient paternal inheritance of what are now known to be Cand . Megaira symbionts (Lee and Kochert 1976 ); later work showed biparental inheritance of Rickettsia in Nephotettix planthoppers (Watanabe et al. 2014 ), and Sodalis glossinidia in tsetse fly hosts (De Vooght et al. 2015 ). Biparental inheritance allows symbionts to drive to high frequency without either conferring a benefit to their host, or exhibiting reproductive parasitism. It also creates an environment where mixed infections become common, which may lead to the evolution of increased virulence (associated with competition for transmission) and also potentiates recombination.
Genomic organisation and interactions with the nuclear genome
Genomic architectural organisation and variation in cies.
Cytoplasmically inherited genomes are highly variable across eukaryotes in terms of both size and structure (Smith and Keeling 2015 ). Mitochondrial and chloroplast genomes are rarely lost entirely (but see (Hjort et al. 2010 ; Keeling 2010 )); however, the diminutive size of mtDNAs in Plasmodium species (~6 kb) (Hikosaka et al. 2011 ) compared to the massive and multi-partite mitochondrial genomes of Silene conica (~11.3 Mb) (Sloan et al. 2012 ) and Larix siberica (~11.7 Mb) (Putintseva et al. 2020 ) highlight the diverse trajectories of cytoplasmic genome evolution following the original endosymbiotic event and subsequent massive transfer to the nucleus prior to the Last Eukaryotic Common Ancestor (Sloan et al. 2018 ).
Plastid genomes are less variable in size than mitochondrial genomes (Wu et al. 2020 ), but non-photosynthetic plastids have seen dramatic reductions in size compared to their photosynthetic ancestors (de Koning and Keeling 2006 ; Barbrook et al. 2014 ). While many CIEs exhibit circular genomes (e.g., most bilaterian mitochondrial genomes (Boore 1999 ) and most eubacterial symbionts), linear (Stampar et al. 2019 ; Escalante et al. 1998 ; Nosek et al. 2004 ; Shao et al. 2009 ), branched (Oldenburg and Bendich 1996 ), and multi-chromosomal arrangements (Wu et al. 2020 ) have arisen multiple separate times across eukaryotes. The absence of Mendelian inheritance in cytoplasmic genomes likely contributes to the tremendous variation observed there, to the extent that once multi-chromosomal genomes evolve, their inheritance is highly fragmented and inconsistent (Wu and Sloan 2019 ).
In parallel, heritable symbiont genomes vary greatly in genome complexity. There is a general pattern of genome reduction in symbiotic microbes, first associated with the transition from environmentally acquired to heritable, and then with the transition from facultative (not required by the host) to obligate (required by the host). Obligately required heritable symbionts have genomes that are commonly <1 Mb, and may be as small as 112 kb (Bennett and Moran 2013 ), in comparison to free-living microbes with genome sizes >4 Mb. Pseudogenization is rapid during the first phases of evolution, often accompanied by proliferation of mobile elements (Bennett and Moran 2013 ). G/C content typically reduces as the genome shrinks, with obligate symbiont genomes typically highly AT rich (Moran et al. 2008 ).
Interactions and molecular cross talk with the nuclear genome
It is common to observe genes in the nuclear genome which have cytoplasmic origin, and transfer of material from both mitochondria and microbial symbionts to the nucleus is ongoing (Bensasson et al. 2001 ; Dunning Hotopp et al. 2007 ). Importantly, recent nuclear derived symbiont sequences on occasion have strong phenotypic effects, potentiating retention (Leclercq et al. 2016 ). In deep evolutionary time, these transfers fuelled the seemingly inevitable gene transfer from the cytoplasm to the nucleus and subsequent genome streamlining that CIEs have repeatedly undergone (Timmis et al. 2004 ; Giannakis et al. 2021 ). This process has resulted in the vast majority of proteins that function in cytoplasmically inherited organelle compartments being encoded by the nucleus (Millar 2007 ; Meisinger et al. 2008 ; van Wijk and Baginsky 2011 ; Muthye and Lavrov 2018 ). The genes and gene products that are still retained in CIE genomes must therefore physically interact with nuclear-encoded gene products. To wit, four of the five multi-subunit enzymes that comprise the electron transport chain and the photosynthetic enzyme complexes of chloroplasts feature intimate interactions between subunits encoded by separately inherited and expressed genomes (Rand et al. 2004 ; Forsythe et al. 2019 ).
Much attention has been paid to the molecular nature of these cytoplasmic-nuclear interactions (Osada and Akashi 2011 ; van der Sluis et al. 2015 ; Beck et al. 2015 ; Adrion et al. 2016 ; Mossman et al. 2017 ; Rand and Mossman 2020 ; Evans et al. 2021 ), but relatively little is known about the stoichiometry of these interactions, except that cytoplasmic gene expression is consistently higher than expression of nuclear-encoded genes involved in the same multi-subunit complexes (Havird and Sloan 2016 ). Further, mitochondrial DNA depletion is associated with a number of different diseases in humans (Blokhin et al. 2008 ; Clay Montier et al. 2009 ; Monickaraj et al. 2012 ; Petersen et al. 2014 ; Pyle et al. 2016 ; Tin et al. 2016 ; Ashar et al. 2017 ; Liu et al. 2020 ), and polyploid plants exhibit elevated cytoplasmic DNA content per cell compared to diploid relatives to maintain cytonuclear stoichiometry following genome doubling (Fernandes Gyorfy et al. 2021 ). Even from this currently limited understanding, it is clear that complex stoichiometric relationships exist between the nuclear and cytoplasmic genomes and gene products, and perturbations to cytonuclear stoichiometry can therefore have drastic consequences for the cells that experience them.
Population and evolutionary genetics of cytoplasmic elements
Mutations and how they spread throughout the cytoplasm.
Cytoplasmically inherited elements present a stark contrast to Mendelian traits as sources of variation. As Bill Birky noted, CIEs are ‘non-stringent’ genetic traits that can vary in quantity as well as sequence – in contrast to Mendelian elements limited to a copy number of two in any diploid cell (Birky 2001 ). Instead, CIEs are often highly multi-copy within cells (Kukat et al. 2011 ; Carelli et al. 2015 ; Schaack et al. 2020 ). As such, the distribution of sequence variation in CIEs is profoundly affected by the distribution of copy number variation, as mutants must first establish within the pool inside a cell, then amongst the cells within an individual, then amongst individuals in the population. Variation in copy number within the cell is also critical to the stoichiometric balance between the cytoplasmic and nuclear genomes, as they contribute to the assembly of the multi-subunit enzyme complexes that carry out bioenergetic processes like photosynthesis and respiration (Forsythe et al. 2019 ).
Mutational spread in cytoplasmically inherited genomes is fundamentally dependent upon the rate of occurrence of new mutations (Sung et al. 2012 ; Waneka et al. 2021 ). However, the multi-copy nature of CIEs makes it practically impossible to determine their absolute mutation rates (Schaack et al. 2020 ). Nevertheless, a large effort across decades has been dedicated to quantifying relative mitochondrial mutation rates and frequency spectra (Brown et al. 1979 ; Wolfe et al. 1987 ; Denver et al. 2000 ; Haag-Liautard et al. 2008 ; Howe et al. 2009 ; Havird and Sloan 2016 ; Allio et al. 2017 ; Konrad et al. 2017 ; Wu et al. 2020 ; Broz et al. 2021 ; Waneka et al. 2021 ), providing valuable information about the extent of heteroplasmy caused by de novo mutations (Waneka et al. 2021 ) and the probability of transmitting those heteroplasmies to the next generation (Konrad et al. 2017 ). Mutational spectra of mtDNA are also important to mutational spread (reviewed in (Katju and Bergthorsson 2019 )). For example, oxidation of guanines (i.e., 8-oxo-G), especially in mitochondria, can result in elevated CG → AT transversions through mispairing with adenine (Cheng et al. 1992 ; Kino et al. 2017 ).
Despite the aforementioned difficulty in ascertaining absolute mutation rates in CIEs, it is clear that CIE mutation rates vary tremendously across taxa. For example, animal mtDNAs exhibit substantially higher mutation rates than plant mtDNAs (Wolfe et al. 1987 ). Indeed, animal mtDNA mutation rate varies more than two orders of magnitude across taxa (Nabholz et al. 2007 ). Certain plant lineages have shown episodic accelerations in cytoplasmic genome mutation rates (Sloan et al. 2014 ; Sloan 2015 ; Havird et al. 2015 ; Williams et al. 2019 ; Broz et al. 2021 ). There is also tremendous variation in mutation rate across different cellular genomes – animal mtDNAs exhibit higher mutation rates than animal nuclear genomes (Brown et al. 1979 ; Wolfe et al. 1987 ; Havird and Sloan 2016 ), but plant nuclear genomes exhibit higher mutation rates than plant cpDNA and plant mtDNAs (Wolfe et al. 1987 ).
Whilst mutation rate is not known for heritable microbes, it is known they vary substantially in substitution rate, with some heritable microbes evolving at a rate comparable to viruses (Gerth et al. 2021 ), and others, like Wolbachia , two-three orders of magnitude slower (Richardson et al. 2012 ). At least some of this variation can be traced back to the different mechanisms of replication and repair across taxa and compartments (Brown et al. 2005 ; Maréchal and Brisson 2010 ; Lewis et al. 2015 ; Gerth et al. 2021 ), all of which have implications for mutation rate (Longley et al. 2005 ; DeBalsi et al. 2017 ; Wu et al. 2020 ).
Mutational masking in cytoplasmic elements
New mutations that arise in CIEs face a dramatically different population genetic landscape compared to Mendelian elements because there are typically many competing cytoplasmic genomes present inside each cell, and because organellar cytoplasmic elements do not experience segregation, as is the case for nuclear genomes during sexual reproduction (Wilton et al. 2018 ). Thus, new mutations face a steep drift barrier, with their effects on host function being masked until reaching higher frequency within a cell or individual (potentially as high as 80% (King and Attardi 1989 ; Boulet et al. 1992 ; Stewart and Chinnery 2015 )). As a consequence, mutations that exist at low frequencies among CIEs in a parent are likely to be lost as a result of the bottleneck that occurs between generations in multicellular organisms.
There are two sides to the mutational masking that results from harbouring many copies of cytoplasmic genomes inside cells: (1) mutations with deleterious fitness effects on the host can persist longer than they otherwise would if maintained in single-copy form within the cell (Otto 2007 ), and (2) mutations with beneficial fitness effects on the host can be lost at higher rates because their effects are largely invisible to selection. Recent high-resolution efforts support the existence of mutational masking, as nonsynonymous mutations are more common and exist at higher frequencies than expected (Waneka et al. 2021 ). Moreover, masking of the fitness effects of mutations is expected to result in a deletion bias (Lawless et al. 2020 ), especially under relaxed selection (Wickett et al. 2008 ), as CIEs with replication advantages (e.g., CIEs with smaller genomes) can rise in frequency within cells rapidly (Wallace 1989 ; Clark et al. 2012 ; Sloan and Wu 2014 ). This latter pattern may contribute to the observation that CIEs exhibit more streamlined genomes compared to their free-living relatives (Timmis et al. 2004 ; Giannakis et al. 2021 ).
The population of CIEs within cells, of cells within tissues, of somatic vs. germ line tissues, and of individual hosts within host populations gives rise to the expectation of multi-level selection, in which elements that have an advantage in terms of spread at a lower level of organisation do not necessarily possess the same advantage at higher levels of organisation (Fig. (Fig.1). 1 ). To wit, mutations that remain at low frequencies across generations in human mtDNA can rise to high frequency in separate tissues within the same individual (Samuels et al. 2013 ; Rebolledo-Jaramillo et al. 2014 ; Li et al. 2015 ). Additionally, recent work in which artificial mixed infections of Buchnera were created within aphids demonstrated a ‘regular winner’, despite strong drift effects - but the winner did not necessarily confer individual level benefits (Perreau et al. 2021 ).
Recombination in the cytoplasm
The misconception that CIEs do not undergo recombination has been largely debunked. For example, phylogenetic and other experimental analyses of animal mitochondrial genomes consistently recover signatures of inter-molecular recombination, indicating that inheritance leakage may play a major role in CIE genome evolution (Mita et al. 1990 ; Kajander et al. 2000 ; Ladoukakis and Zouros 2001 ; Ladoukakis and Eyre-Walker 2004 ; Piganeau et al. 2004 ; Barr et al. 2005 ; Ciborowski et al. 2007 ; Ma and O’Farrell 2015 ; Leducq et al. 2017 ; Dahal et al. 2018 ). Plant plastids and plant mitochondria exhibit recombination-directed repair (Cerutti et al. 1995 ; Day and Madesis 2007 ; Maréchal and Brisson 2010 ; Davila et al. 2011 ; Gualberto and Newton 2017 ; Chevigny et al. 2020 ; Wu et al. 2020 ), as well as rampant structural rearrangement via repeat-mediated recombination (Palmer 1983 ; Ogihara et al. 1988 ; Palmer and Herbon 1988 ; Gray et al. 1999 ; Arrieta-Montiel and Mackenzie 2011 ; Cole et al. 2018 ; Wu and Sloan 2019 ; Xia et al. 2020 ). This latter phenomenon, termed substoichiometric shifting, makes for extensive, but heritable structural variation within individuals (Woloszynska 2009 ; Maréchal and Brisson 2010 ; Arrieta-Montiel and Mackenzie 2011 ; Davila et al. 2011 ).
Recombination has played such a large role in plant CIEs that their relatively slow rate of molecular evolution is thought to be due, at least in part, to recombination (Palmer and Herbon 1988 ; Chevigny et al. 2020 ; Wu et al. 2020 ), as heteroplasmies may be eliminated from intracellular populations via gene conversion. Whether the occasional and episodic accelerations in rates of cytoplasmic genome evolution in some plant lineages (Williams et al. 2019 ; Broz et al. 2021 ) is associated with altered recombinatorial activity remains an open question. Heritable microbes also show clear signatures of recombination (Baldo et al. 2006 ), as well as acquisition of genetic material from other bacteria (Nikoh et al. 2014 ), which commonly involves phage transfer (Kaur et al. 2021 ; Boyd et al. 2021 ).
The evolutionary consequences of cytoplasmic recombination are profound: recombination can act as a barrier to new mutations through gene conversion and can facilitate the rise of beneficial mutations and the elimination of deleterious mutations by separating those mutations from their genomic backgrounds (Neiman and Taylor 2009 ), particularly when distinct CIEs occur inside the same cell or organism.
Cytoplasmic adaptation
The seemingly asexual nature of animal cytoplasmic genomes suggests that they, like other asexual elements, will experience impaired adaptive evolution. Experimental evidence, however, has found this not to be the case, as numerous studies have reported signatures of positive selection acting within the mitochondrial genome (Mishmar et al. 2003 ; Ruiz-Pesini et al. 2004 ; Meiklejohn et al. 2007 ). Furthermore, clinal patterns in mtDNA genomes have been detected across several species, indicating signatures of adaptation (Camus et al. 2017 ; Silva et al. 2014 ). Early studies by Lynch and Blanchard ( 1998 ) found that mitochondrial genes had higher ratios of nonsynonymous to synonymous mutations in relation to the nuclear genome of plants, invertebrate and fungal taxa (Lynch and Blanchard 1998 ). Most recently, Morales et al. ( 2015 ) found evidence for positive selection on several amino acids in the mtDNA of the Australian eastern yellow robin ( Eopsaltria australis ) populations. The authors additionally found nuclear genome homogeneity within the robin populations sampled indicating that there were high levels of gene flow, thus the signatures of positive selection were unique to the mtDNA (Morales et al. 2015 ). The combined outcomes of these studies suggest that certain mtDNA protein-coding genes of natural populations might well have been shaped by positive selection.
Less evidence is available linking chloroplast genomes to adaptive processes, but this could be because of the slower rates of evolution, higher levels of complexity or the fact that dissecting the contributions of multiple organelle genomes is complicated. Nevertheless, there has been some work in domesticated species testing these questions. For example, research on rice ( Oryza ) has identified 14 chloroplast genes with strong signatures of positive selection, with these genes being mainly related to photosynthetic function. Interestingly, authors found that eight of these genes were independently found in sun-loving species, whereas other photosynthetic genes were selected in shade-tolerating species (Gao et al. 2019 ). Other studies have directly examined the effects of cytonuclear interactions across two different ecological environments. The sunflower genus Helianthus is commonly used as a model as many of its species have adapted to very distinct niches (Levin 2003 ). Sambatti and colleagues performed reciprocal transplant experiments between H. annus and H. petiolaris which inhabit mesic and xeric habitats respectively (Sambatti et al. 2008 ). In addition to examining both coevolved strains, authors used all possible backcross combinations to dissect the contribution of the cytoplasm and nuclear genome, finding that the cytoplasm was the main driver for fitness, and is therefore adapted to these two contrasting environments (Sambatti et al. 2008 ).
Heritable symbionts have been commonly observed to be under strong selection. Invasion of heritable symbionts into populations in real time has been observed on numerous occasions. For instance, a classic example is the wave of Wolbachia that induced cytoplasmic incompatibility (CI) which swept through Californian D. simulans populations in the 1980s (Turelli and Hoffmann 1991 ). Similarly, heritable Spiroplasma that provide tolerance to nematode parasitism have spread through North America, and Rickettsia spreading through whitefly populations has been observed over the last 20 years (Himler et al. 2011 ; Shi et al. 2021 ). It is also common to observe that symbionts either themselves have low diversity - or that associated mitochondria have low diversity - implying a recent history of joint selection. Finally, it is notable that heritable microbes (unlike mitochondria and chloroplasts) may segregate during host reproduction, with a fraction of progeny not inheriting them. Their maintenance thus requires some form of drive - either a benefit to host survival or reproductive parasitism. As such, it is argued they are never neutral traits, but are maintained by a selection - segregational loss balance (Jaenike 2012 ). In addition, they present different modularities of adaptive variation - like other CIEs they have different circulating variants in a population, but in addition, these commonly exist alongside uninfected cytotypes, which are the equivalent of a null allele.
Coadaptation
Coadaptation with nuclear encoded proteins and systems.
The transfer of genetic material from cytoplasmic elements to the nucleus is thought to have created strong pressures for both nuclear and cytoplasmic genomes to cooperate with one another. Excessive amounts of conflict can have severe consequences to both host and symbiont. One of the classic demonstrations of mitonuclear coadaptation comes from studies using hybrid crosses from natural populations. The copepod species Tigriopus californicus has become one of the main wild model systems, primarily due to the high level of intraspecific divergence in mtDNA genomes. While crosses between populations give F1 offspring with normal (if not elevated) fitness compared to the parental generation, there is a drastic decrease in fitness in the F2 generations and beyond (Burton 1990 ). Using backcrossing approaches, they discovered that this decrease in fitness was caused by severe mitonuclear incompatibilities (Burton and Lee 1994 ), and the proportion of the maternal nuclear genome appears to be positively correlated with developmental rate in backcrossed individuals (Han and Barreto 2021 ). The nuclear-encoded mitochondrial genes (those interacting with genes encoded in the mtDNA) of the T. californicus genome have also been shown to coadapt with mtDNA, exhibiting elevated mutation-rate-corrected rates of evolution (i.e., d N /d S ) compared to the rest of the nuclear-encoded DNA, matching the rapid pace of evolution in their mtDNA counterparts (Barreto et al. 2018 ).
Similarly, chloroplast genomes are predicted to be under strong selection to coadapt, and much work has been done to document the effects of plastid-nuclear interactions on plant fitness (Greiner et al. 2011 ; Postel and Touzet 2020 ). Nearly all of the ~75–80 proteins encoded by the chloroplast genome are involved in protein complexes which exhibit important functions that are essential to plant function, such as Rubisco and photosystems I and II. One of the best examples of coadaptation between chloroplast and nuclear genomes after intraspecific hybridisation comes from the genus Oenothera (Stubbe 1989 ; Greiner and Bock 2013 ), in which three basic haploid nuclear genomes can be paired with five different chloroplast genomes; giving a total of 30 possible chloro-nuclear combinations. Of these, only 12 produce a viable green phenotype, whereas the 18 remaining associations lead to various degrees of cytonuclear incompatibilities, from reduced phenotypic capacity to embryo lethality (Cleland 1972 ; Dietrich et al. 1997 ). Subsequent work suggests that the radiation within Oenothera is approximately 1 million years old, suggesting that incompatibilities and coadaptation mechanisms have rapidly evolved (Greiner et al. 2008 ). Although most chloroplast genomes evolve relatively slowly, occasional accelerations in evolutionary rate have occurred throughout angiosperms (Williams et al. 2019 ). In these cases, the nuclear-encoded interacting partners of chloroplast-encoded proteins exhibit corresponding increases in evolutionary rate, reflecting the co-evolutionary dynamics of plastid-nuclear interactions (Bock et al. 2014 ; Zhang et al. 2015 ; Dai et al. 2016 ; Weng et al. 2016 ; Rockenbach et al. 2016 ; Havird et al. 2017 ; Li et al. 2019 ; Forsythe et al. 2021 ).
Heritable symbionts are distinct from organelles in their interaction with the host in that symbiont proteomes are generally considered to be encoded within their genomes, rather than jointly with the nuclear genome. Thus, this route to coadaptation is less important. Nevertheless, symbionts have profound interplay with their host in terms of cell biology, organismal development, physiology and anatomy. In particular, many heritable symbionts form obligate partnerships with their host - such that neither party can live alone. For beneficial symbionts, there are systems to control symbiont number through antimicrobial production (Login et al. 2011 ), development of specific systems for housing and transmitting symbionts, alongside membrane systems/transporters for metabolite exchange (Feng et al. 2019 ), which all represent adaptations on the host to house and maintain symbiosis. On the symbiont side, there is loss of cell walls and pathways required for growth outside of the host environment, leading to reliance on host supply of nutrients. An interesting phenomenon is dependence - where the host cannot live without the symbiont for reasons other than the services supplied by the symbiont. One of the first cases of hereditary microbial symbiosis - between bacteria and plants of the family Rubiaceae - is one of these, where loss of the symbiont was observed to impede host development (Miller 1990 ). Further cases include the requirement of Asobara tabida for a particular strain of Wolbachia to complete oogenesis (Dedeine et al. 2001 ). These cases likely represent the host evolving around the presence/products of the symbiont, such that symbiont removal results in loss of function.
Coadaptation across the cytoplasm
The study of cytoplasmically inherited agents has acknowledged the diversity of genetic material in the cytoplasm, but rarely examined interactions between the parties. Interactions may be either direct (e.g., a mito-symbiont interaction), or indirect (an evolutionary response in one that impacts the other through coinheritance). Whilst little is known about the former, evidence of indirect impacts is plentiful - selection on one party feeds through to the other inherited elements. For example, the spread of Wolbachia causing CI through a population carries the linked mtDNA haplotype, and the selective sweep reduces mtDNA diversity at the population level (Turelli et al. 1992 ; Hurst and Jiggins 2005 ; Deng et al. 2021 ). Indeed, there are a variety of cases where symbionts are thought to have driven the movement of mtDNA across species boundaries (Turelli et al. 1992 ; Hurst and Jiggins 2005 ; Deng et al. 2021 ). Following spread, the presence of a symbiont at equilibrium in the population has been considered to reduce the effective population of mtDNA to that associated with the fraction which carries the symbiont, conceptually equivalent to background selection removing certain individuals from the pool of individuals out of which mutations arise and spread. More recently, it has been argued the reciprocal pattern is also likely - selective sweeps on mtDNA impact diversity (and indeed frequency and presence) of symbionts (Fenton et al. 2021 ). Thus, the diversity and population genetics of the cytoplasm should be taken summatively, rather than simply with regard to individual elements.
Conflicts between cytoplasmically inherited elements and their hosts
Maternal inheritance produces an association between symbiont fitness and that of their female, but not male host. This has two primary consequences - the mother’s curse (selection optimises CIEs to female phenotype fitness) and reproductive parasitism (selection optimises CIEs to maximise the production and survival of infected daughters).
Mother’s curse
The theoretical framework for the mother’s curse hypothesis was first described in the 1990s (Frank and Hurst 1996 ), with further theoretical support proposed a decade later. This framework is simple; the uniparental maternal inheritance of mtDNA means that males are prone to inherit mutations that are selected through the female lineage, even if these mutations are detrimental to males. Consequently, males are expected to accumulate these sexually antagonistic mutations over evolutionary time (Fig. (Fig.2A 2A ).

Differences in inheritance patterns between nuclear and cytoplasmic elements provides an arena for intergenomic conflict. A Mothers curse hypothesis: maternal inheritance of mitochondria can result in the accumulation of mutations with sexually antagonistic effects in the mtDNA genome. B Cytoplasmic sex ratio distortion in species with separate sexes. Commonly, investment into male and female offspring is equal. Maternal inheritance ties symbiont fitness to the production and survival of female hosts. This is manifested in parthenogenesis induction (left), where all progeny are daughters. Male-killing (middle), where the symbiont kills male progeny it enters, and sibling females have greater access to resources, and higher survival, as a result. Feminisation (right), where the symbiont impacts development in progeny that have a male karyotype such that they differentiate as female hosts. C Cytoplasmic incompatibility is the result of severe miscommunication between cytoplasmic and nuclear genomes, and a classic example of how cytoplasmic elements can spread through a population. When hosts carrying the symbiont (red) mate with uninfected hosts (grey), CI can result in inviable offspring in a unidrectional (left) or bidirectional (right) fashion.
The first experimental evidence for mother’s curse came from a Drosophila study that examined the effects of mtDNA genetic variation on the transcriptomic response (Innocenti et al. 2011 ). This study used cybrids (cytoplasmic hybrids), in which the mitochondrial genomes from five fly strains sourced from different parts of the world were coupled independently to an isogenic nuclear background, decoupling the effects of mtDNA from those of the nuclear genome. Approximately 10% of the nuclear genome was differentially expressed in males, whereas only a handful of genes were affected in females. Interestingly, these differentially expressed genes were particularly involved in the male reproductive system (testes, accessory glands, ejaculatory duct). Another clear example of mother’s curse came from humans in which a male-biased mutation in the mtDNA resulted in Leber’s hereditary optical neuropathy (Milot et al. 2017 ). The particular mutation was tracked over a 290-year period, by identifying via genealogical records that it was first recorded in Canada from a woman arriving from France in the 1600s. Given the large male fitness consequences conferred by the mutation, natural selection would be expected to remove this variant from the population, but authors noticed a slight increase in frequency, suggesting a female fitness benefit (Milot et al. 2017 ). More recently though, the scope of mother’s curse has broadened to not only include reproductive traits, but also other sex-specific life-history traits (Montooth and Dhawanjewar 2019 ; Nagarajan-Radha et al. 2020 ; Carnegie et al. 2021 ).
What is not currently clear is the extent to which mother’s curse impacts maternally inherited elements beyond mitochondria. Conceptually, beneficial heritable symbioses are expected to experience a parallel process in terms of adaptation - the capacity of a maternally inherited symbiont to protect a male host, for instance, derives solely from correlated selection from its impact on female hosts. However, the degree to which maternally inherited beneficial symbioses perform less well in male hosts has not been investigated.
Reproductive parasitism: Investment into female over male progeny and gametes
Both symbionts and mitochondria are known to bias the pattern of host investment into, and survival of, female hosts/gametes over male (see (Hurst and Frost 2015 ) for review). In hermaphroditic plants, mitochondrial variants impact the development of anthers and pollen formation in the phenotype of cytoplasmic male sterility (CMS). These variants divert resources to reproduction through ovule/seed, and in so doing, promote their own transmission (Fig. (Fig.2B 2B ).
In arthropods, symbionts variously induce parthenogenetic reproduction (thus ensuring all progeny are female and can transmit the element), feminise hosts that are otherwise ‘programmed’ to male development, or selectively kill male hosts they enter. This last phenotype appears to be spiteful, but is actually an adaptive phenotype when the death of male hosts releases resources directly (through consumption) or indirectly (through relaxed competition) to sibling females (Hurst and Majerus 1993 ). In ladybirds, for instance, dead male eggs (through which the symbiont cannot be transmitted) are consumed by their sisters (which carry the symbiont and can transmit them). Embryonic male-killing in dioecious species is thus conceptually equivalent to CMS in hermaphrodites, as a source of resource reallocation from male to female reproduction. Male-killing may also occur later in development, and here it is commonly associated with infectious transmission of symbionts from male hosts, with the symbiont showing mixed modes of transmission (maternal inheritance through females, infectious transmission through males (Hurst 1991 ).
The sex ratio/allocation distorting phenotypes described above have impacts on the individual, but also strong ecological and evolutionary consequences. Parthenogenesis inducing symbionts can spread to fixation, converting the species from sexual to asexual (Stouthamer et al. 1990 ). Cytoplasmic male sterility, feminisation and male-killing agents can cause strongly female-biased population sex ratios, and these may alter patterns of sexual selection (Jiggins et al. 2000 ; Charlat et al. 2007 ) and indeed the capacity of a host to effectively reproduce (Dyson and Hurst 2004 ). Perhaps most importantly, they engender strong selection on their hosts to restore sex allocation/sex ratio to parity. This is reflected in nuclear restorer genes against mitochondria inducing CMS (Frank 1989 ), and suppressor genes rescuing male function against male-killers (Hornett et al. 2006 ) or preventing transmission of feminizers (Rigaud and Juchault 1992 ). The strongly female-biased sex ratios created when cytoplasmic sex-ratio distorters are common creates intense Fisherian selection for suppression/restorer elements, such that the spread of suppression/restorer genes represents some of the strongest selective events recorded in natural populations (e.g., (Charlat et al. 2007 ; Hornett et al. 2014 )). Further, the evolution of suppression may hide the underlying reproductive parasitism, which may only become apparent in crosses between populations (Hornett et al. 2006 ), hybridisation (Frank 1989 ), or for symbionts, transinfection to a novel host species (Sasaki et al. 2002 ). Indeed, the commonness with which CMS suppression evolves is reflected in the emergence of CMS in about 20% of hybridisation events in plants (Frank 1989 ).
There are several open questions in our understanding of sex ratio distortion. In terms of incidence, mitochondrial sex allocation distorters are commonly observed in plants, but not in animals. This may be associated with differences in coding capacity/genetics of plant vs. animal genomes (mutational constraint). Symbionts that distort sex ratio/allocation are very commonly observed in arthropods, and have been hypothesised as present in sea urchins (Carrier et al. 2021 ). Given heritable microbes are common, it is expected that sex ratio distortion would be present in a wider array of host than is currently recognised. In terms of impact, a key emerging question is the nature of restorer and suppression mutations. What are the host systems that are impacted in this co-evolutionary arms race? It has been widely hypothesised these may involve modifications of the sex determination system, as alterations of the signal or target of the symbiont (Hornett et al. 2014 ). This awaits further discovery of the mechanism of male-killing and of suppression; however, that symbionts alter splicing of key sex determination genes like doublesex supports sex determination as a focus for suppression (Sugimoto et al. 2010 ).
Reproductive parasitism: Cytoplasmic incompatibility
Cytoplasmic Incompatibility (CI) phenotypes describe the failure of zygote development where the male parent is infected with a symbiont and the female parent either does not have that symbiont, or carries a different strain of the symbiont. Originally described as a phenotype of the Wolbachia in arthropods (Yen and Barr 1971 ), this phenotype has since been associated with diverse insect symbionts, including Cardinium and Rickettsiella (Hunter et al. 2003 ; Rosenwald et al. 2020 ). The symbionts conferring CI spread as it imposes a cost solely on uninfected lineages; the positive frequency-dependent nature of the advantage means invasion either requires the symbiont to reach a threshold frequency, or has an alternate phenotype that allows initial establishment. In contrast to symbionts with sex ratio distorting phenotypes, the symbiont becomes less costly to the host when it is common, simply because symbiont-infected females are unaffected. Indeed, hosts are selected to retain the symbiont to provide immunity against CI when the symbiont is common (Fig. (Fig.2C 2C ).
Cytoplasmic Incompatibility is a very important phenotype for two reasons. First, it can be applied in the form of release of infected males to suppress target vector populations, and in the form of inoculative release of strains that combine CI and suppress viral replication, to reduce vectorial capacity. Thus, basic research on cytoplasmic symbionts (Hedges et al. 2008 ; Teixeira et al. 2008 ) has translated into applied public health protection measures (Utarini et al. 2021 ). Second, spread of a CI inducing symbiont in one population or species may provide a unidirectional barrier to hybridisation against another, and the spread of distinct strains may produce a bidirectional barrier (Bordenstein et al. 2001 ). In both cases, the symbiont spread induces reproductive isolation and thus potentiates speciation.
Conclusions
In 1919, Thomas Hunt Morgan in his Principles of Heredity wrote:
‘That there may be substances in the cytoplasm that propagate themselves and are outside the influence of the nucleus, must, of course, be at once conceded as possible despite the fact that, aside from certain plastids, all the Mendelian evidence fails to show that there are such characters’. (Morgan 1919 )
By contrast, the past 100 years have created awareness of the vast amount of genetic biodiversity found inhabiting the cytoplasm. Very early on it was noted that these genetic elements did not follow Mendelian inheritance patterns, with inheritance being mostly maternal. One topic that remains a long-standing and unresolved question are why most organelle genomes transmit maternally? Since the initial observations of CIEs, many exceptions to this rule have been discovered, from paternal inheritance of mtDNA in cucumbers, to doubly-uniparental inheritance in bivalves to biparental inheritance in yeast, and symbionts combining infectious and vertical transmission. Still, a predominance for cytoplasmic elements to be inherited via the maternal lineage is evident from the phylogenetic record. The tight association of cytoplasmic genomes with the rest of the cell, plus the non-Mendelian inheritance patterns of CIEs results in fascinating co-evolutionary dynamics that manifest at multiple scales of biological organisation. Thus, the diversity, inheritance, and functional roles of CIEs across eukaryotes remain an important and open question in biology, with fundamental implications for the cells and organisms in which they reside.
Supplementary information
Acknowledgements.
We thank three anonymous reviewers who made very helpful suggestions to improve this manuscript.
Author contributions
All authors contributed to the ideas presented and writing of the manuscript.
MFC was funded by a UKRI Fellowship (NE/V014307/1) and a Leverhulme Trust grant (RPG-2019-109). JS was funded by the National Science Foundation (DEB – 1753695, DEB – 1753851, IOS – 1829176, IOS – 2145811). BAL and JS were funded by the New Mexico Institute of Mining and Technology. GDDH was funded by a UKRI grant (NE/S012346/1).
Competing interests
The authors declare no competing interests.
Associate editor: Barbara Mable.
Publisher’s note Springer Nature remains neutral with regard to jurisdictional claims in published maps and institutional affiliations.
The online version contains supplementary material available at 10.1038/s41437-022-00540-2.
- Adrion JR, White PS, Montooth KL. The roles of compensatory evolution and constraint in aminoacyl tRNA synthetase evolution. Mol Biol Evol. 2016; 33 :152–161. [ PMC free article ] [ PubMed ] [ Google Scholar ]
- Allio R, Donega S, Galtier N, Nabholz B. Large variation in the ratio of mitochondrial to nuclear mutation rate across animals: implications for genetic diversity and the use of mitochondrial DNA as a molecular marker. Mol Biol Evol. 2017; 34 :2762–2772. [ PubMed ] [ Google Scholar ]
- Allison TM, Radzvilavicius AL, Dowling DK. Selection for biparental inheritance of mitochondria under hybridization and mitonuclear fitness interactions. Proc Biol Sci. 2021; 288 :20211600. [ PMC free article ] [ PubMed ] [ Google Scholar ]
- Arrieta-Montiel MP, Mackenzie SA. Plant mitochondrial genomes and recombination. In: Kempken F, editor. Plant Mitochondria. New York, NY: Springer New York; 2011. pp. 65–82. [ Google Scholar ]
- Ashar FN, Zhang Y, Longchamps RJ, Lane J, Moes A, Grove ML, et al. Association of mitochondrial DNA copy number with cardiovascular disease. JAMA Cardiol. 2017; 2 :1247–1255. [ PMC free article ] [ PubMed ] [ Google Scholar ]
- Baldo L, Bordenstein S, Wernegreen JJ, Werren JH. Widespread recombination throughout W olbachia genomes. Mol Biol Evol. 2006; 23 :437–449. [ PubMed ] [ Google Scholar ]
- Barbrook AC, Voolstra CR, Howe CJ. The chloroplast genome of a Symbiodinium sp. clade C3 isolate. Protist. 2014; 165 :1–13. [ PubMed ] [ Google Scholar ]
- Barr CM, Neiman M, Taylor DR. Inheritance and recombination of mitochondrial genomes in plants, fungi and animals. N. Phytol. 2005; 168 :39–50. [ PubMed ] [ Google Scholar ]
- Barreto FS, Watson ET, Lima TG, Willett CS, Edmands S, Li W, et al. Genomic signatures of mitonuclear coevolution across populations of Tigriopus californicus . Nat Ecol Evolution. 2018; 2 :1250–1257. [ PubMed ] [ Google Scholar ]
- Baur E. Das Wesen und die Erblichkeitsverhältnisse der „Varietates albomarginatae hort.“ vonPelargonium zonale. Z für Indukt Abstamm- und Vererbungslehre. 1908; 1 :330–351. [ Google Scholar ]
- Beck EA, Thompson AC, Sharbrough J, Brud E, Llopart A. Gene flow between Drosophila yakuba and Drosophila santomea in subunit V of cytochrome c oxidase: A potential case of cytonuclear cointrogression. Evolution. 2015; 69 :1973–1986. [ PMC free article ] [ PubMed ] [ Google Scholar ]
- Bennett GM, Moran NA. Small, smaller, smallest: the origins and evolution of ancient dual symbioses in a Phloem-feeding insect. Genome Biol Evol. 2013; 5 :1675–1688. [ PMC free article ] [ PubMed ] [ Google Scholar ]
- Bensasson D, Zhang D-X, Hartl DL, Hewitt GM. Mitochondrial pseudogenes: evolution’s misplaced witnesses. Trends Ecol Evol. 2001; 16 :314–321. [ PubMed ] [ Google Scholar ]
- Bentley KE, Mandel JR, McCauley DE (2010) Paternal leakage and heteroplasmy of mitochondrial genomes in Silene vulgari s: evidence from experimental crosses. Genetics 185:961–968 [ PMC free article ] [ PubMed ]
- Birky CW., Jr The inheritance of genes in mitochondria and chloroplasts: laws, mechanisms, and models. Annu Rev Genet. 2001; 35 :125–148. [ PubMed ] [ Google Scholar ]
- Birky CW, Strausberg RL, Forster JL, Perlman PS. Vegetative segregation of mitochondria in yeast: Estimating parameters using a random model. Mol Gen Genet. 1978; 158 :251–261. [ Google Scholar ]
- Blokhin A, Vyshkina T, Komoly S, Kalman B. Variations in mitochondrial DNA copy numbers in MS brains. J Mol Neurosci. 2008; 35 :283–287. [ PubMed ] [ Google Scholar ]
- Bock DG, Andrew RL, Rieseberg LH. On the adaptive value of cytoplasmic genomes in plants. Mol Ecol. 2014; 23 :4899–4911. [ PubMed ] [ Google Scholar ]
- Boore JL. Animal mitochondrial genomes. Nucleic Acids Res. 1999; 27 :1767–1780. [ PMC free article ] [ PubMed ] [ Google Scholar ]
- Bordenstein SR, O’Hara FP, Werren JH. Wolbachia -induced incompatibility precedes other hybrid incompatibilities in Nasonia . Nature. 2001; 409 :707–710. [ PubMed ] [ Google Scholar ]
- Boulet L, Karpati G, Shoubridge EA. Distribution and threshold expression of the tRNA(Lys) mutation in skeletal muscle of patients with myoclonic epilepsy and ragged-red fibers (MERRF) Am J Hum Genet. 1992; 51 :1187–1200. [ PMC free article ] [ PubMed ] [ Google Scholar ]
- Boyd BM, Chevignon G, Patel V, Oliver KM, Strand MR (2021) Evolutionary genomics of APSE: a tailed phage that lysogenically converts the bacterium Hamiltonella defensa into a heritable protective symbiont of aphids. Virol J 18:219 [ PMC free article ] [ PubMed ]
- Breton S, Beaupré HD, Stewart DT, Hoeh WR, Blier PU. The unusual system of doubly uniparental inheritance of mtDNA: isn’t one enough? Trends Genet. 2007; 23 :465–474. [ PubMed ] [ Google Scholar ]
- Breton S, Burger G, Stewart DT, Blier PU. Comparative analysis of gender-associated complete mitochondrial genomes in marine mussels (Mytilus spp.) Genetics. 2006; 172 :1107–1119. [ PMC free article ] [ PubMed ] [ Google Scholar ]
- Brown TA, Cecconi C, Tkachuk AN, Bustamante C, Clayton DA. Replication of mitochondrial DNA occurs by strand displacement with alternative light-strand origins, not via a strand-coupled mechanism. Genes Dev. 2005; 19 :2466–2476. [ PMC free article ] [ PubMed ] [ Google Scholar ]
- Brown WM, George M, Jr, Wilson AC. Rapid evolution of animal mitochondrial DNA. Proc Natl Acad Sci USA. 1979; 76 :1967–1971. [ PMC free article ] [ PubMed ] [ Google Scholar ]
- Broz AK, Waneka G, Wu Z, Fernandes Gyorfy M, Sloan DB (2021) Detecting de novo mitochondrial mutations in angiosperms with highly divergent evolutionary rates. Genetics 218:iyab039 [ PMC free article ] [ PubMed ]
- Burton RS (1990) Hybrid breakdown in developmental time in the copepod Tigriopus californicus . Evolution 44:1814–1822 [ PubMed ]
- Burton RS, Lee BN. Nuclear and mitochondrial gene genealogies and allozyme polymorphism across a major phylogeographic break in the copepod Tigriopus californicus . Proc Natl Acad Sci USA. 1994; 91 :5197–5201. [ PMC free article ] [ PubMed ] [ Google Scholar ]
- Burzyński A, Zbawicka M, Skibinski DOF, Wenne R. Evidence for recombination of mtDNA in the marine mussel Mytilus trossulus from the Baltic. Mol Biol Evol. 2003; 20 :388–392. [ PubMed ] [ Google Scholar ]
- Camus MF, Wolff JN, Sgrò CM, Dowling DK. Experimental support that natural selection has shaped the latitudinal distribution of mitochondrial haplotypes in Australian Drosophila melanogaster . Mol Biol Evol. 2017; 34 :2600–2612. [ PubMed ] [ Google Scholar ]
- Carelli V, Maresca A, Caporali L, Trifunov S, Zanna C, Rugolo M. Mitochondria: Biogenesis and mitophagy balance in segregation and clonal expansion of mitochondrial DNA mutations. Int J Biochem Cell Biol. 2015; 63 :21–24. [ PubMed ] [ Google Scholar ]
- Carnegie L, Reuter M, Fowler K, Lane N, Camus MF. Mother’s curse is pervasive across a large mitonuclear Drosophila panel. Evol Lett. 2021; 5 :230–239. [ PMC free article ] [ PubMed ] [ Google Scholar ]
- Carrier TJ, Leigh BA, Deaker DJ, Devens HR, Wray GA, Bordenstein SR et al. (2021) Microbiome reduction and endosymbiont gain from a switch in sea urchin life history. Proc Natl Acad Sci USA 118:e2022023118 [ PMC free article ] [ PubMed ]
- Cato SA, Richardson TE (1996) Inter- and intraspecific polymorphism at chloroplast SSR loci and the inheritance of plastids in Pinus radiata D. Don. Theor Appl Genet 93:587–592 [ PubMed ]
- Cerutti H, Johnson AM, Boynton JE, Gillham NW. Inhibition of chloroplast DNA recombination and repair by dominant negative mutants of Escherichia coli RecA. Mol Cell Biol. 1995; 15 :3003–3011. [ PMC free article ] [ PubMed ] [ Google Scholar ]
- Charlat S, Hornett EA, Fullard JH, Davies N, Roderick GK, Wedell N, et al. Extraordinary flux in sex ratio. Science. 2007; 317 :214. [ PubMed ] [ Google Scholar ]
- Charlat S, Reuter M, Dyson EA, Hornett EA, Duplouy A, Davies N, et al. Male-killing bacteria trigger a cycle of increasing male fatigue and female promiscuity. Curr Biol. 2007; 17 :273–277. [ PubMed ] [ Google Scholar ]
- Cheng KC, Cahill DS, Kasai H, Nishimura S, Loeb LA. 8-Hydroxyguanine, an abundant form of oxidative DNA damage, causes G-T and A-C substitutions. J Biol Chem. 1992; 267 :166–172. [ PubMed ] [ Google Scholar ]
- Chevigny N, Schatz-Daas D, Lotfi F, Gualberto JM (2020) DNA repair and the stability of the plant mitochondrial genome. Int J Mol Sci 21:328 [ PMC free article ] [ PubMed ]
- Chiu W-L, Stubbe W, Sears BB. Plastid inheritance in Oenothera : organelle genome modifies the extent of biparental plastid transmission. Curr Genet. 1988; 13 :181–189. [ Google Scholar ]
- Christie JR, Beekman M. Selective sweeps of mitochondrial DNA can drive the evolution of uniparental inheritance. Evolution. 2017; 71 :2090–2099. [ PubMed ] [ Google Scholar ]
- Christie JR, Schaerf TM, Beekman M. Selection against heteroplasmy explains the evolution of uniparental inheritance of mitochondria. PLoS Genet. 2015; 11 :e1005112. [ PMC free article ] [ PubMed ] [ Google Scholar ]
- Ciborowski KL, Consuegra S, García de Leániz C, Beaumont MA, Wang J, Jordan WC. Rare and fleeting: an example of interspecific recombination in animal mitochondrial DNA. Biol Lett. 2007; 3 :554–557. [ PMC free article ] [ PubMed ] [ Google Scholar ]
- Clark KA, Howe DK, Gafner K, Kusuma D, Ping S, Estes S, et al. Selfish little circles: transmission bias and evolution of large deletion-bearing mitochondrial DNA in Caenorhabditis briggsae nematodes. PLoS One. 2012; 7 :e41433. [ PMC free article ] [ PubMed ] [ Google Scholar ]
- Clay Montier LL, Deng JJ, Bai Y. Number matters: control of mammalian mitochondrial DNA copy number. J Genet Genomics. 2009; 36 :125–131. [ PMC free article ] [ PubMed ] [ Google Scholar ]
- Cleland RE (1963) The cytogenetics of Oenothera . Adv Genet 11:147–237
- Cole LW, Guo W, Mower JP, Palmer JD. High and variable rates of repeat-mediated mitochondrial genome rearrangement in a genus of plants. Mol Biol Evol. 2018; 35 :2773–2785. [ PubMed ] [ Google Scholar ]
- Correns C. Zur kenntnis der rolle von kern und plasma bei der vererbung. Z Indukt Abstamm Vererbungsl. 1909; 2 :331–340. [ Google Scholar ]
- Dahal S, Dubey S, Raghavan SC. Homologous recombination-mediated repair of DNA double-strand breaks operates in mammalian mitochondria. Cell Mol Life Sci. 2018; 75 :1641–1655. [ PubMed ] [ Google Scholar ]
- Dai B, Guo H, Huang C, Zhang X, Lin Z (2016) Genomic heterozygosity and hybrid breakdown in cotton ( Gossypium ): different traits, different effects. BMC Genet 17:58 [ PMC free article ] [ PubMed ]
- Davila JI, Arrieta-Montiel MP, Wamboldt Y, Cao J, Hagmann J, Shedge V et al. (2011) Double-strand break repair processes drive evolution of the mitochondrial genome in Arabidopsis . BMC Biol 9:64 [ PMC free article ] [ PubMed ]
- Day A, Madesis P. DNA replication, recombination, and repair in plastids. In: Bock R, editor. Cell and Molecular Biology of Plastids. Berlin, Heidelberg: Springer Berlin Heidelberg; 2007. pp. 65–119. [ Google Scholar ]
- De Vooght L, Caljon G, Van Hees J, Van Den Abbeele J. Paternal transmission of a secondary symbiont during mating in the viviparous tsetse fly. Mol Biol Evol. 2015; 32 :1977–1980. [ PMC free article ] [ PubMed ] [ Google Scholar ]
- DeBalsi KL, Hoff KE, Copeland WC. Role of the mitochondrial DNA replication machinery in mitochondrial DNA mutagenesis, aging and age-related diseases. Ageing Res Rev. 2017; 33 :89–104. [ PMC free article ] [ PubMed ] [ Google Scholar ]
- Dedeine F, Vavre F, Fleury F, Loppin B, Hochberg ME, Bouletreau M (2001) Removing symbiotic Wolbachia bacteria specifically inhibits oogenesis in a parasitic wasp. Proc Natl Acad Sci USA 98:6247–6252 [ PMC free article ] [ PubMed ]
- Deng J, Assandri G, Chauhan P, Futahashi R, Galimberti A, Hansson B et al. (2021) Wolbachia -driven selective sweep in a range expanding insect species. BMC Ecol Evol 21:181 [ PMC free article ] [ PubMed ]
- Denver DR, Morris K, Lynch M, Vassilieva LL, Thomas WK (2000) High direct estimate of the mutation rate in the mitochondrial genome of Caenorhabditis elegans . Science 289:2342–2344 [ PubMed ]
- Dietrich W, Wagner WL, Raven PH (1997) Systematics of Oenothera section Oenothera subsection Oenothera ( Onagraceae ). Syst Bot Monogr 50:1
- Douglas AE. Host benefit and the evolution of specialization in symbiosis. Heredity. 1998; 81 :599–603. [ Google Scholar ]
- Drew GC, Budge GE, Frost CL, Neumann P, Siozios S, Yañez O, et al. Transitions in symbiosis: evidence for environmental acquisition and social transmission within a clade of heritable symbionts. ISME J. 2021; 15 :2956–2968. [ PMC free article ] [ PubMed ] [ Google Scholar ]
- Dunning Hotopp JC, Clark ME, Oliveira DCSG, Foster JM, Fischer P, Muñoz Torres MC, et al. Widespread lateral gene transfer from intracellular bacteria to multicellular eukaryotes. Science. 2007; 317 :1753–1756. [ PubMed ] [ Google Scholar ]
- Dyson EA, Hurst GDD. Persistence of an extreme sex-ratio bias in a natural population. Proc Natl Acad Sci USA. 2004; 101 :6520–6523. [ PMC free article ] [ PubMed ] [ Google Scholar ]
- Escalante AA, Freeland DE, Collins WE, Lal AA. The evolution of primate malaria parasites based on the gene encoding cytochrome b from the linear mitochondrial genome. Proc Natl Acad Sci USA. 1998; 95 :8124–8129. [ PMC free article ] [ PubMed ] [ Google Scholar ]
- Evans BJ, Peter BM, Melnick DJ, Andayani N, Supriatna J, Zhu J et al. (2021) Mitonuclear interactions and introgression genomics of macaque monkeys ( Macaca ) highlight the influence of behaviour on genome evolution. Proc Biol Sci 288:20211756 [ PMC free article ] [ PubMed ]
- Fauré S, Noyer JL, Carreel F, Horry JP, Bakry F, Lanaud C (1994) Maternal inheritance of chloroplast genome and paternal inheritance of mitochondrial genome in bananas ( Musa acuminata ). Curr Genet 25:265–269 [ PubMed ]
- Feng H, Edwards N, Anderson CMH, Althaus M, Duncan RP, Hsu Y-C et al. (2019) Trading amino acids at the aphid– Buchnera symbiotic interface. Proc Natl Acad Sci USA 116:16003–16011 [ PMC free article ] [ PubMed ]
- Fenton A, Camus MF, Hurst GDD. Positive selection on mitochondria may eliminate heritable microbes from arthropod populations. Proc Biol Sci. 2021; 288 :20211735. [ PMC free article ] [ PubMed ] [ Google Scholar ]
- Fernandes Gyorfy M, Miller ER, Conover JL, Grover CE, Wendel JF, Sloan DB et al. (2021) Nuclear-cytoplasmic balance: whole genome duplications induce elevated organellar genome copy number. Plant J 108:219–230 [ PubMed ]
- Fontaine KM, Cooley JR, Simon C (2007) Evidence for paternal leakage in hybrid periodical cicadas ( Hemiptera: Magicicada spp.). PLoS One 2:e892 [ PMC free article ] [ PubMed ]
- Forsythe ES, Sharbrough J, Havird JC, Warren JM, Sloan DB (2019) CyMIRA: The cytonuclear molecular interactions reference for Arabidopsis . Genome Biol Evol 11:2194–2202 [ PMC free article ] [ PubMed ]
- Forsythe ES, Williams AM, Sloan DB. Genome-wide signatures of plastid-nuclear coevolution point to repeated perturbations of plastid proteostasis systems across angiosperms. Plant Cell. 2021; 33 :980–997. [ PMC free article ] [ PubMed ] [ Google Scholar ]
- Frank SA. The evolutionary dynamics of cytoplasmic male sterility. Am Nat. 1989; 133 :345–376. [ Google Scholar ]
- Frank SA, Hurst LD. Mitochondria and male disease. Nature. 1996; 383 :224. [ PubMed ] [ Google Scholar ]
- Gao L-Z, Liu Y-L, Zhang D, Li W, Gao J, Liu Y et al. (2019) Evolution of Oryza chloroplast genomes promoted adaptation to diverse ecological habitats. Commun Biol 2:278 [ PMC free article ] [ PubMed ]
- Gerth M, Martinez-Montoya H, Ramirez P, Masson F, Griffin JS, Aramayo R et al. (2021) Rapid molecular evolution of Spiroplasma symbionts of Drosophila . Microb Genom 7:000503 [ PMC free article ] [ PubMed ]
- Ghiselli F, Milani L (2020) Linking the mitochondrial genotype to phenotype: a complex endeavour. Phil Trans Roy Soc B, 375:20190169 [ PMC free article ] [ PubMed ]
- Giannakis K, Arrowsmith SJ, Richards L, Gasparini S (2021) Universal features shaping organelle gene retention. bioRxiv
- Gray MW, Burger G, Lang BF. Mitochondrial evolution. Science. 1999; 283 :1476–1481. [ PubMed ] [ Google Scholar ]
- Greiner S, Bock R. Tuning a ménage à trois: Co-evolution and co-adaptation of nuclear and organellar genomes in plants. BioEssays. 2013; 35 :354–365. [ PubMed ] [ Google Scholar ]
- Greiner S, Rauwolf U, Meurer J, Herrmann RG. The role of plastids in plant speciation. Mol Ecol. 2011; 20 :671–691. [ PubMed ] [ Google Scholar ]
- Greiner S, Wang X, Rauwolf U, Silber MV, Mayer K, Meurer J et al. (2008) The complete nucleotide sequences of the five genetically distinct plastid genomes of Oenothera , subsection Oenothera : I. Sequence evaluation and plastome evolution †. Nucleic Acids Res 36:2366–2378 [ PMC free article ] [ PubMed ]
- Gualberto JM, Newton KJ. Plant mitochondrial genomes: dynamics and mechanisms of mutation. Annu Rev Plant Biol. 2017; 68 :225–252. [ PubMed ] [ Google Scholar ]
- Gyllensten U, Wharton D, Josefsson A, Wilson AC. Paternal inheritance of mitochondrial DNA in mice. Nature. 1991; 352 :255–257. [ PubMed ] [ Google Scholar ]
- Haag-Liautard C, Coffey N, Houle D, Lynch M, Charlesworth B, Keightley PD (2008) Direct estimation of the mitochondrial DNA mutation rate in Drosophila melanogaster . PLoS Biol 6:e204 [ PMC free article ] [ PubMed ]
- Hadjivasiliou Z, Pomiankowski A, Seymour RM, Lane N. Selection for mitonuclear co-adaptation could favour the evolution of two sexes. Proc Biol Sci. 2012; 279 :1865–1872. [ PMC free article ] [ PubMed ] [ Google Scholar ]
- Han K-L, Barreto FS (2021) Pervasive mitonuclear coadaptation underlies fast development in interpopulation hybrids of a marine crustacean. Genome Biol Evol 13:evab004 [ PMC free article ] [ PubMed ]
- Hartmann AC, Baird AH, Knowlton N, Huang D. The paradox of environmental symbiont acquisition in obligate mutualisms. Curr Biol. 2017; 27 :3711–3716. [ PubMed ] [ Google Scholar ]
- Havey MJ. Predominant paternal transmission of the mitochondrial genome in cucumber. J Hered. 1997; 88 :232–235. [ Google Scholar ]
- Havey MJ, McCreight JD, Rhodes B, Taurick G (1998) Differential transmission of the Cucumis organellar genomes. Theor Appl Genet 97:122–128
- Havird JC, Sloan DB. The roles of mutation, selection, and expression in determining relative rates of evolution in mitochondrial versus nuclear genomes. Mol Biol Evol. 2016; 33 :3042–3053. [ PMC free article ] [ PubMed ] [ Google Scholar ]
- Havird JC, Trapp P, Miller CM, Bazos I, Sloan DB. Causes and consequences of rapidly evolving mtDNA in a plant lineage. Genome Biol Evol. 2017; 9 :323–336. [ PMC free article ] [ PubMed ] [ Google Scholar ]
- Havird JC, Whitehill NS, Snow CD, Sloan DB. Conservative and compensatory evolution in oxidative phosphorylation complexes of angiosperms with highly divergent rates of mitochondrial genome evolution. Evolution. 2015; 69 :3069–3081. [ PMC free article ] [ PubMed ] [ Google Scholar ]
- Hedges LM, Brownlie JC, O’Neill SL, Johnson KN (2008) Wolbachia and virus protection in insects. Science 322:702 [ PubMed ]
- Hikosaka K, Watanabe Y-I, Kobayashi F, Waki S, Kita K, Tanabe K (2011) Highly conserved gene arrangement of the mitochondrial genomes of 23 Plasmodium species. Parasitol Int 60:175–180 [ PubMed ]
- Hill WG, Robertson A. The effect of linkage on limits to artificial selection. Genetical Res. 1966; 8 :269–294. [ PubMed ] [ Google Scholar ]
- Himler AG, Adachi-Hagimori T, Bergen JE, Kozuch A, Kelly SE, Tabashnik BE, et al. Rapid spread of a bacterial symbiont in an invasive whitefly is driven by fitness benefits and female bias. Science. 2011; 332 :254–256. [ PubMed ] [ Google Scholar ]
- Hjort K, Goldberg AV, Tsaousis AD, Hirt RP, Embley TM. Diversity and reductive evolution of mitochondria among microbial eukaryotes. Philos Trans R Soc Lond B Biol Sci. 2010; 365 :713–727. [ PMC free article ] [ PubMed ] [ Google Scholar ]
- Hornett EA, Charlat S, Duplouy AMR, Davies N, Roderick GK, Wedell N, et al. Evolution of male-killer suppression in a natural population. PLoS Biol. 2006; 4 :e283. [ PMC free article ] [ PubMed ] [ Google Scholar ]
- Hornett EA, Moran B, Reynolds LA, Charlat S, Tazzyman S, Wedell N et al. (2014) The evolution of sex ratio distorter suppression affects a 25 cM genomic region in the butterfly Hypolimnas bolina . PLoS Genet 10:e1004822 [ PMC free article ] [ PubMed ]
- Howe DK, Baer CF, Denver DR (2009) High rate of large deletions in Caenorhabditis briggsae mitochondrial genome mutation processes. Genome Biol Evol 2:29–38 [ PMC free article ] [ PubMed ]
- Hunter MS, Perlman SJ, Kelly SE (2003) A bacterial symbiont in the Bacteroidetes induces cytoplasmic incompatibility in the parasitoid wasp Encarsia pergandiella . Proc Biol Sci 270:2185–2190 [ PMC free article ] [ PubMed ]
- Hurst LD. Parasite diversity and the evolution of diploidy, multicellularity and anisogamy. J Theor Biol. 1990; 144 :429–443. [ PubMed ] [ Google Scholar ]
- Hurst LD. The incidences and evolution of cytoplasmic male killers. Proc R Soc Lond Ser B: Biol Sci. 1991; 244 :91–99. [ Google Scholar ]
- Hurst GDD, Frost CL. Reproductive parasitism: maternally inherited symbionts in a biparental world. Cold Spring Harb Perspect Biol. 2015; 7 :a017699. [ PMC free article ] [ PubMed ] [ Google Scholar ]
- Hurst LD, Hamilton WD. Cytoplasmic fusion and the nature of sexes. Proc R Soc Lond Ser B: Biol Sci. 1992; 247 :189–194. [ Google Scholar ]
- Hurst GDD, Jiggins FM. Problems with mitochondrial DNA as a marker in population, phylogeographic and phylogenetic studies: the effects of inherited symbionts. Proc Biol Sci. 2005; 272 :1525–1534. [ PMC free article ] [ PubMed ] [ Google Scholar ]
- Hurst GDD, Majerus MEN. Why do maternally inherited microorganisms kill males? Heredity. 1993; 71 :81–95. [ Google Scholar ]
- Innocenti P, Morrow EH, Dowling DK. Experimental evidence supports a sex-specific selective sieve in mitochondrial genome evolution. Science. 2011; 332 :845–848. [ PubMed ] [ Google Scholar ]
- Jaenike J. Population genetics of beneficial heritable symbionts. Trends Ecol Evol. 2012; 27 :226–232. [ PubMed ] [ Google Scholar ]
- Jiggins FM, Hurst GD, Majerus ME (2000) Sex-ratio-distorting Wolbachia causes sex-role reversal in its butterfly host. Proc Biol Sci 267:69–73 [ PMC free article ] [ PubMed ]
- Kajander OA, Rovio AT, Majamaa K, Poulton J, Spelbrink JN, Holt IJ, et al. Human mtDNA sublimons resemble rearranged mitochondrial genomes found in pathological states. Hum Mol Genet. 2000; 9 :2821–2835. [ PubMed ] [ Google Scholar ]
- Katju V, Bergthorsson U. Old trade, new tricks: insights into the spontaneous mutation process from the partnering of classical mutation accumulation experiments with high-throughput genomic approaches. Genome Biol Evol. 2019; 11 :136–165. [ PMC free article ] [ PubMed ] [ Google Scholar ]
- Kaur R, Shropshire JD, Cross KL, Leigh B, Mansueto AJ, Stewart V et al. (2021) Living in the endosymbiotic world of Wolbachia : A centennial review. Cell Host Microbe 29:879–893 [ PMC free article ] [ PubMed ]
- Keeling PJ. The endosymbiotic origin, diversification and fate of plastids. Philos Trans R Soc Lond B Biol Sci. 2010; 365 :729–748. [ PMC free article ] [ PubMed ] [ Google Scholar ]
- King MP, Attardi G. Human cells lacking mtDNA: repopulation with exogenous mitochondria by complementation. Science. 1989; 246 :500–503. [ PubMed ] [ Google Scholar ]
- Kino K, Hirao-Suzuki M, Morikawa M, Sakaga A, Miyazawa H. Generation, repair and replication of guanine oxidation products. Genes Environ. 2017; 39 :21. [ PMC free article ] [ PubMed ] [ Google Scholar ]
- de Koning AP, Keeling PJ (2006) The complete plastid genome sequence of the parasitic green alga Helicosporidium sp. is highly reduced and structured. BMC Biol 4:12 [ PMC free article ] [ PubMed ]
- Konrad A, Thompson O, Waterston RH, Moerman DG, Keightley PD, Bergthorsson U et al. (2017) Mitochondrial mutation rate, spectrum and heteroplasmy in Caenorhabditis elegans spontaneous mutation accumulation lines of differing population size. Mol Biol Evol 34:1319–1334 [ PMC free article ] [ PubMed ]
- Kukat C, Wurm CA, Spåhr H, Falkenberg M, Larsson N-G, Jakobs S. Super-resolution microscopy reveals that mammalian mitochondrial nucleoids have a uniform size and frequently contain a single copy of mtDNA. Proc Natl Acad Sci USA. 2011; 108 :13534–13539. [ PMC free article ] [ PubMed ] [ Google Scholar ]
- Kvist L, Martens J, Nazarenko AA, Orell M (2003) Paternal leakage of mitochondrial DNA in the great tit ( Parus major ). Mol Biol Evol 20:243–247 [ PubMed ]
- Ladoukakis ED, Eyre-Walker A. Evolutionary genetics: Direct evidence of recombination in human mitochondrial DNA. Heredity. 2004; 93 :321–321. [ PubMed ] [ Google Scholar ]
- Ladoukakis ED, Zouros E. Recombination in animal mitochondrial DNA: evidence from published sequences. Mol Biol Evol. 2001; 18 :2127–2131. [ PubMed ] [ Google Scholar ]
- Ladoukakis ED, Zouros E (2017) Evolution and inheritance of animal mitochondrial DNA: rules and exceptions. J Biol Res-Thessaloniki 24:1–7 [ PMC free article ] [ PubMed ]
- Lawless C, Greaves L, Reeve AK, Turnbull DM, Vincent AE. The rise and rise of mitochondrial DNA mutations. Open Biol. 2020; 10 :200061. [ PMC free article ] [ PubMed ] [ Google Scholar ]
- Leclercq S, Thézé J, Chebbi MA, Giraud I, Moumen B, Ernenwein L et al. (2016) Birth of a W sex chromosome by horizontal transfer of Wolbachia bacterial symbiont genome. Proc Natl Acad Sci USA 113:15036–15041 [ PMC free article ] [ PubMed ]
- Lederberg J. Cell genetics and hereditary symbiosis. Physiol Rev. 1952; 32 :403–430. [ PubMed ] [ Google Scholar ]
- Leducq J-B, Henault M, Charron G, Nielly-Thibault L, Terrat Y, Fiumera HL, et al. Mitochondrial recombination and introgression during speciation by hybridization. Mol Biol Evol. 2017; 34 :1947–1959. [ PMC free article ] [ PubMed ] [ Google Scholar ]
- Lee W-SB, Kochert G (1976) Bacterial endosymbionts in Volvox carteri ( Chlorophyceae )1. J Phycol 12:194–197
- Leeks A, Dos Santos M, West SA. Transmission, relatedness, and the evolution of cooperative symbionts. J Evol Biol. 2019; 32 :1036–1045. [ PMC free article ] [ PubMed ] [ Google Scholar ]
- Levin DA. The cytoplasmic factor in plant speciation. sbot. 2003; 28 :5–11. [ Google Scholar ]
- Lewis SC, Joers P, Willcox S, Griffith JD, Jacobs HT, Hyman BC (2015) A rolling circle replication mechanism produces multimeric lariats of mitochondrial DNA in Caenorhabditis elegans . PLoS Genet 11:e1004985 [ PMC free article ] [ PubMed ]
- Li M, Schröder R, Ni S, Madea B, Stoneking M. Extensive tissue-related and allele-related mtDNA heteroplasmy suggests positive selection for somatic mutations. Proc Natl Acad Sci USA. 2015; 112 :2491–2496. [ PMC free article ] [ PubMed ] [ Google Scholar ]
- Li C, Sun X, Conover JL, Zhang Z, Wang J, Wang X et al. (2019) Cytonuclear coevolution following homoploid hybrid speciation in Aegilops tauschii . Mol Biol Evol 36:341–349 [ PMC free article ] [ PubMed ]
- Liu X, Longchamps RJ, Wiggins K, Raffield LM, Bielak LF, Zhao W et al. (2020) Association of mitochondrial DNA copy number with cardiometabolic diseases in a large cross-sectional study of multiple ancestries. medRxiv [ PMC free article ] [ PubMed ]
- Login FH, Balmand S, Vallier A, Vincent-Monégat C, Vigneron A, Weiss-Gayet M, et al. Antimicrobial peptides keep insect endosymbionts under control. Science. 2011; 334 :362–365. [ PubMed ] [ Google Scholar ]
- Longley MJ, Graziewicz MA, Bienstock RJ, Copeland WC. Consequences of mutations in human DNA polymerase gamma. Gene. 2005; 354 :125–131. [ PubMed ] [ Google Scholar ]
- Lynch M, Blanchard JL. Deleterious mutation accumulation in organelle genomes. Genetica. 1998; 102–103 :29–39. [ PubMed ] [ Google Scholar ]
- Ma H, O’Farrell PH (2015) Selections that isolate recombinant mitochondrial genomes in animals. Elife 4:e07247 [ PMC free article ] [ PubMed ]
- Macey JR, Pabinger S, Barbieri CG, Buring ES, Gonzalez VL, Mulcahy DG, et al. Evidence of two deeply divergent co-existing mitochondrial genomes in the Tuatara reveals an extremely complex genomic organization. Commun Biol. 2021; 4 :116. [ PMC free article ] [ PubMed ] [ Google Scholar ]
- Magalon H, Nidelet T, Martin G, Kaltz O. Host growth conditions influence experimental evolution of life history and virulence of a parasite with vertical and horizontal transmission. Evolution. 2010; 64 :2126–2138. [ PubMed ] [ Google Scholar ]
- Maréchal A, Brisson N. Recombination and the maintenance of plant organelle genome stability. N. Phytol. 2010; 186 :299–317. [ PubMed ] [ Google Scholar ]
- Meiklejohn CD, Montooth KL, Rand DM. Positive and negative selection on the mitochondrial genome. Trends Genet. 2007; 23 :259–263. [ PubMed ] [ Google Scholar ]
- Meisinger C, Sickmann A, Pfanner N. The mitochondrial proteome: from inventory to function. Cell. 2008; 134 :22–24. [ PubMed ] [ Google Scholar ]
- Millar AH. The plant mitochondrial proteome. In: Šamaj J, Thelen JJ, editors. Plant Proteomics. Berlin, Heidelberg: Springer Berlin Heidelberg; 2007. pp. 226–246. [ Google Scholar ]
- Miller IM. Bacterial leaf nodule symbiosis. In: Callow JA, editor. Advances in Botanical Research. Academic Press; 1990. pp. 163–234. [ Google Scholar ]
- Milot E, Moreau C, Gagnon A, Cohen AA, Brais B, Labuda D. Mother’s curse neutralizes natural selection against a human genetic disease over three centuries. Nat Ecol Evol. 2017; 1 :1400–1406. [ PubMed ] [ Google Scholar ]
- Mishmar D, Ruiz-Pesini E, Golik P, Macaulay V, Clark AG, Hosseini S, et al. Natural selection shaped regional mtDNA variation in humans. Proc Natl Acad Sci USA. 2003; 100 :171–176. [ PMC free article ] [ PubMed ] [ Google Scholar ]
- Mita S, Rizzuto R, Moraes CT, Shanske S, Arnaudo E, Fabrizi GM, et al. Recombination via flanking direct repeats is a major cause of large-scale deletions of human mitochondrial DNA. Nucleic Acids Res. 1990; 18 :561–567. [ PMC free article ] [ PubMed ] [ Google Scholar ]
- Monickaraj F, Aravind S, Gokulakrishnan K, Sathishkumar C, Prabu P, Prabu D, et al. Accelerated aging as evidenced by increased telomere shortening and mitochondrial DNA depletion in patients with type 2 diabetes. Mol Cell Biochem. 2012; 365 :343–350. [ PubMed ] [ Google Scholar ]
- Montooth KL, Dhawanjewar AS (2019) Temperature-sensitive reproduction and the physiological and evolutionary potential for Mother’s Curse. Integr Comp Biol 59:890–899 [ PMC free article ] [ PubMed ]
- Morales HE, Pavlova A, Joseph L, Sunnucks P. Positive and purifying selection in mitochondrial genomes of a bird with mitonuclear discordance. Mol Ecol. 2015; 24 :2820–2837. [ PubMed ] [ Google Scholar ]
- Moran NA, McCutcheon JP, Nakabachi A. Genomics and evolution of heritable bacterial symbionts. Annu Rev Genet. 2008; 42 :165–190. [ PubMed ] [ Google Scholar ]
- Morgan TH (1919) The physical basis of heredity. J. B. Lippincott Co.
- Mossman JA, Tross JG, Jourjine NA, Li N, Wu Z, Rand DM (2017) Mitonuclear interactions mediate transcriptional responses to hypoxia in Drosophila . Mol Biol Evol 34:447–466 [ PMC free article ] [ PubMed ]
- Muthye V, Lavrov DV. Characterization of mitochondrial proteomes of nonbilaterian animals. IUBMB Life. 2018; 70 :1289–1301. [ PubMed ] [ Google Scholar ]
- Nabholz B, Glémin S, Galtier N. Strong variations of mitochondrial mutation rate across mammals—the longevity hypothesis. Mol Biol Evol. 2007; 25 :120–130. [ PubMed ] [ Google Scholar ]
- Nagarajan-Radha V, Aitkenhead I, Clancy DJ, Chown SL, Dowling DK (2020) Sex-specific effects of mitochondrial haplotype on metabolic rate in Drosophila melanogaster support predictions of the Mother’s Curse hypothesis. Philos Trans R Soc Lond B Biol Sci 375:20190178 [ PMC free article ] [ PubMed ]
- Neale DB, Marshall KA, Sederoff RR (1989) Chloroplast and mitochondrial DNA are paternally inherited in Sequoia sempervirens D. Don Endl. Proc Natl Acad Sci USA 86:9347–9349 [ PMC free article ] [ PubMed ]
- Neale DB, Sederoff RR. Paternal inheritance of chloroplast DNA and maternal inheritance of mitochondrial DNA in loblolly pine. Theor Appl Genet. 1989; 77 :212–216. [ PubMed ] [ Google Scholar ]
- Neiman M, Taylor DR. The causes of mutation accumulation in mitochondrial genomes. Proc Biol Sci. 2009; 276 :1201–1209. [ PMC free article ] [ PubMed ] [ Google Scholar ]
- Nikoh N, Hosokawa T, Moriyama M, Oshima K, Hattori M, Fukatsu T (2014) Evolutionary origin of insect– Wolbachia nutritional mutualism. Proc Natl Acad Sci USA 111:10257–10262 [ PMC free article ] [ PubMed ]
- Nissanka N, Moraes CT. Mitochondrial DNA heteroplasmy in disease and targeted nuclease-based therapeutic approaches. EMBO Rep. 2020; 21 :e49612. [ PMC free article ] [ PubMed ] [ Google Scholar ]
- Nosek J, Novotna M, Hlavatovicova Z, Ussery DW, Fajkus J, Tomaska L (2004) Complete DNA sequence of the linear mitochondrial genome of the pathogenic yeast Candida parapsilosis . Mol Genet Genomics 272:173–180 [ PubMed ]
- Nunes MDS, Dolezal M, Schlötterer C (2013) Extensive paternal mtDNA leakage in natural populations of Drosophila melanogaster . Mol Ecol 22:2106–2117 [ PMC free article ] [ PubMed ]
- Ogihara Y, Terachi T, Sasakuma T. Intramolecular recombination of chloroplast genome mediated by short direct-repeat sequences in wheat species. Proc Natl Acad Sci USA. 1988; 85 :8573–8577. [ PMC free article ] [ PubMed ] [ Google Scholar ]
- Oldenburg DJ, Bendich AJ (1996) Size and structure of replicating mitochondrial DNA in cultured tobacco cells. Plant Cell 8:447–461 [ PMC free article ] [ PubMed ]
- Osada N, Akashi H. Mitochondrial–nuclear interactions and accelerated compensatory evolution: evidence from the primate cytochrome C oxidase complex. Mol Biol Evol. 2011; 29 :337–346. [ PubMed ] [ Google Scholar ]
- Otto SP. The evolutionary consequences of polyploidy. Cell. 2007; 131 :452–462. [ PubMed ] [ Google Scholar ]
- Palmer JD. Chloroplast DNA exists in two orientations. Nature. 1983; 301 :92–93. [ Google Scholar ]
- Palmer JD, Herbon LA. Plant mitochondrial DNA evolved rapidly in structure, but slowly in sequence. J Mol Evol. 1988; 28 :87–97. [ PubMed ] [ Google Scholar ]
- Passamonti M, Boore JL, Scali V (2003) Molecular evolution and recombination in gender-associated mitochondrial DNAs of the Manila clam Tapes philippinarum . Genetics 164:603–611 [ PMC free article ] [ PubMed ]
- Passamonti M, Ghiselli F. Doubly uniparental inheritance: two mitochondrial genomes, one precious model for organelle DNA inheritance and evolution. DNA Cell Biol. 2009; 28 :79–89. [ PubMed ] [ Google Scholar ]
- Perreau J, Zhang B, Maeda GP, Kirkpatrick M, Moran NA (2021) Strong within-host selection in a maternally inherited obligate symbiont: Buchnera and aphids. Proc Natl Acad Sci USA 118:e2102467118 [ PMC free article ] [ PubMed ]
- Petersen MH, Budtz-Jørgensen E, Sørensen SA, Nielsen JE, Hjermind LE, Vinther-Jensen T, et al. Reduction in mitochondrial DNA copy number in peripheral leukocytes after onset of Huntington’s disease. Mitochondrion. 2014; 17 :14–21. [ PubMed ] [ Google Scholar ]
- Piganeau G, Gardner M, Eyre-Walker A. A broad survey of recombination in animal mitochondria. Mol Biol Evolution. 2004; 21 :2319–2325. [ PubMed ] [ Google Scholar ]
- Postel Z, Touzet P (2020) Cytonuclear genetic incompatibilities in plant speciation. Plants 9:487 [ PMC free article ] [ PubMed ]
- Putintseva YA, Bondar EI, Simonov EP, Sharov VV, Oreshkova NV, Kuzmin DA et al. (2020) Siberian larch ( Larix sibirica Ledeb.) mitochondrial genome assembled using both short and long nucleotide sequence reads is currently the largest known mitogenome. BMC Genomics 21:654 [ PMC free article ] [ PubMed ]
- Pyle A, Anugrha H, Kurzawa-Akanbi M, Yarnall A, Burn D, Hudson G. Reduced mitochondrial DNA copy number is a biomarker of Parkinson’s disease. Neurobiol Aging. 2016; 38 :216.e7–216.e10. [ PMC free article ] [ PubMed ] [ Google Scholar ]
- Radzvilavicius AL, Hadjivasiliou Z, Pomiankowski A, Lane N. Selection for mitochondrial quality drives evolution of the germline. PLoS Biol. 2016; 14 :e2000410. [ PMC free article ] [ PubMed ] [ Google Scholar ]
- Rand DM, Haney RA, Fry AJ. Cytonuclear coevolution: the genomics of cooperation. Trends Ecol Evol. 2004; 19 :645–653. [ PubMed ] [ Google Scholar ]
- Rand DM, Mossman JA. Mitonuclear conflict and cooperation govern the integration of genotypes, phenotypes and environments. Philos Trans R Soc Lond B Biol Sci. 2020; 375 :20190188. [ PMC free article ] [ PubMed ] [ Google Scholar ]
- Rebolledo-Jaramillo B, Su MS-W, Stoler N, McElhoe JA, Dickins B, Blankenberg D, et al. Maternal age effect and severe germ-line bottleneck in the inheritance of human mitochondrial DNA. Proc Natl Acad Sci USA. 2014; 111 :15474–15479. [ PMC free article ] [ PubMed ] [ Google Scholar ]
- Richardson MF, Weinert LA, Welch JJ, Linheiro RS, Magwire MM, Jiggins FM et al. (2012) Population genomics of the Wolbachia endosymbiont in Drosophila melanogaster . PLoS Genet 8:e1003129 [ PMC free article ] [ PubMed ]
- Rigaud T, Juchault P (1992) Genetic control of the vertical transmission of a cytoplasmic sex factor in Armadillidium vulgare Latr. (Crustacea, Oniscidea). Heredity 68:47–52
- Rockenbach K, Havird JC, Monroe JG, Triant DA, Taylor DR, Sloan DB. Positive selection in rapidly evolving plastid-nuclear enzyme complexes. Genetics. 2016; 204 :1507–1522. [ PMC free article ] [ PubMed ] [ Google Scholar ]
- Roossinck MJ. Lifestyles of plant viruses. Philos Trans R Soc Lond B Biol Sci. 2010; 365 :1899–1905. [ PMC free article ] [ PubMed ] [ Google Scholar ]
- Rosenwald LC, Sitvarin MI, White JA (2020) Endosymbiotic Rickettsiella causes cytoplasmic incompatibility in a spider host. Proc Biol Sci 287:20201107 [ PMC free article ] [ PubMed ]
- Ross JA, Howe DK, Coleman-Hulbert A, Denver DR, Estes S (2016) Paternal mitochondrial transmission in intra-species Caenorhabditis briggsae hybrids. Mol Biol Evol 33:3158–3160 [ PMC free article ] [ PubMed ]
- Ruiz-Pesini E, Mishmar D, Brandon M, Procaccio V, Wallace DC. Effects of purifying and adaptive selection on regional variation in human mtDNA. Science. 2004; 303 :223–226. [ PubMed ] [ Google Scholar ]
- Sagan L. On the origin of mitosing cells. J Theor Biol. 1967; 14 :255–274. [ PubMed ] [ Google Scholar ]
- Sambatti JBM, Ortiz-Barrientos D, Baack EJ, Rieseberg LH. Ecological selection maintains cytonuclear incompatibilities in hybridizing sunflowers. Ecol Lett. 2008; 11 :1082–1091. [ PMC free article ] [ PubMed ] [ Google Scholar ]
- Samuels DC, Li C, Li B, Song Z, Torstenson E, Boyd Clay H, et al. Recurrent tissue-specific mtDNA mutations are common in humans. PLoS Genet. 2013; 9 :e1003929. [ PMC free article ] [ PubMed ] [ Google Scholar ]
- Sasaki T, Kubo T, Ishikawa H (2002) Interspecific transfer of Wolbachia between two lepidopteran insects expressing cytoplasmic incompatibility: a Wolbachia variant naturally infecting Cadra cautella causes male killing in Ephestia kuehniella . Genetics 162:1313–1319 [ PMC free article ] [ PubMed ]
- Sato M, Sato K. Maternal inheritance of mitochondrial DNA by diverse mechanisms to eliminate paternal mitochondrial DNA. Biochim Biophys Acta. 2013; 1833 :1979–1984. [ PubMed ] [ Google Scholar ]
- Sato K, Sato M. Multiple ways to prevent transmission of paternal mitochondrial DNA for maternal inheritance in animals. J Biochem. 2017; 162 :247–253. [ PubMed ] [ Google Scholar ]
- Schaack S, Ho EKH, Macrae F. Disentangling the intertwined roles of mutation, selection and drift in the mitochondrial genome. Philos Trans R Soc Lond B Biol Sci. 2020; 375 :20190173. [ PMC free article ] [ PubMed ] [ Google Scholar ]
- Schneider A, Stelljes C, Adams C, Kirchner S, Burkhard G, Jarzombski S et al. (2015) Low frequency paternal transmission of plastid genes in Brassicaceae . Transgenic Res 24:267–277 [ PubMed ]
- Shao R, Kirkness EF, Barker SC. The single mitochondrial chromosome typical of animals has evolved into 18 minichromosomes in the human body louse, Pediculus humanus. Genome Res. 2009; 19 :904–912. [ PMC free article ] [ PubMed ] [ Google Scholar ]
- Shi P-Q, Chen X-Y, Chen X-S, Lv N, Liu Y, Qiu B-L (2021) Rickettsia increases its infection and spread in whitefly populations by manipulating the defense patterns of the host plant. FEMS Microbiol Ecol 97:fiab032 [ PubMed ]
- Silva G, Lima FP, Martel P, Castilho R. Thermal adaptation and clinal mitochondrial DNA variation of European anchovy. Proc Biol Sci. 2014; 281 :20141093. [ PMC free article ] [ PubMed ] [ Google Scholar ]
- Skibinski DO, Gallagher C, Beynon CM (1994) Sex-limited mitochondrial DNA transmission in the marine mussel Mytilus edulis . Genetics 138:801–809 [ PMC free article ] [ PubMed ]
- Sloan DB. Using plants to elucidate the mechanisms of cytonuclear co-evolution. N. Phytol. 2015; 205 :1040–1046. [ PubMed ] [ Google Scholar ]
- Sloan DB, Alverson AJ, Chuckalovcak JP, Wu M, McCauley DE, Palmer JD, et al. Rapid evolution of enormous, multichromosomal genomes in flowering plant mitochondria with exceptionally high mutation rates. PLoS Biol. 2012; 10 :e1001241. [ PMC free article ] [ PubMed ] [ Google Scholar ]
- Sloan DB, Triant DA, Forrester NJ, Bergner LM, Wu M, Taylor DR (2014) A recurring syndrome of accelerated plastid genome evolution in the angiosperm tribe Sileneae ( Caryophyllaceae ). Mol Phylogenet Evol 72:82–89 [ PubMed ]
- Sloan DB, Warren JM, Williams AM, Wu Z, Abdel-Ghany SE, Chicco AJ, et al. Cytonuclear integration and co-evolution. Nat Rev Genet. 2018; 19 :635–648. [ PMC free article ] [ PubMed ] [ Google Scholar ]
- Sloan DB, Wu Z. History of plastid DNA insertions reveals weak deletion and at mutation biases in angiosperm mitochondrial genomes. Genome Biol Evol. 2014; 6 :3210–3221. [ PMC free article ] [ PubMed ] [ Google Scholar ]
- van der Sluis EO, Bauerschmitt H, Becker T, Mielke T, Frauenfeld J, Berninghausen O, et al. Parallel structural evolution of mitochondrial ribosomes and OXPHOS complexes. Genome Biol Evol. 2015; 7 :1235–1251. [ PMC free article ] [ PubMed ] [ Google Scholar ]
- Smith DR, Keeling PJ. Mitochondrial and plastid genome architecture: Reoccurring themes, but significant differences at the extremes. Proc Natl Acad Sci USA. 2015; 112 :10177–10184. [ PMC free article ] [ PubMed ] [ Google Scholar ]
- Stampar SN, Broe MB, Macrander J, Reitzel AM, Daly M (2019) Linear mitochondrial genome in anthozoa ( Cnidaria ): A case study in Ceriantharia . Sci Rep. 9:6094 [ PMC free article ] [ PubMed ]
- Stewart DT, Breton S, Blier PU, Hoeh WR (2009) Masculinization events and doubly uniparental inheritance of mitochondrial DNA: A model for understanding the evolutionary dynamics of gender-associated mtDNA in mussels. In Evolutionary Biology. Springer, Berlin, Heidelberg, 163–173
- Stewart JB, Chinnery PF. The dynamics of mitochondrial DNA heteroplasmy: implications for human health and disease. Nat Rev Genet. 2015; 16 :530–542. [ PubMed ] [ Google Scholar ]
- Stouthamer R, Luck RF, Hamilton WD (1990) Antibiotics cause parthenogenetic Trichogramma ( Hymenoptera/Trichogrammatidae ) to revert to sex. Proc Natl Acad Sci USA 87:2424–2427 [ PMC free article ] [ PubMed ]
- Stubbe W. Oenothera—An ideal system for studying the interactions of genome and plastome. Plant Mol Biol Rep. 1989; 7 :245–257. [ Google Scholar ]
- Sugimoto TN, Fujii T, Kayukawa T, Sakamoto H, Ishikawa Y (2010) Expression of a doublesex homologue is altered in sexual mosaics of Ostrinia scapulalis moths infected with Wolbachia . Insect Biochem Mol Biol 40:847–854 [ PubMed ]
- Sung W, Ackerman MS, Miller SF, Doak TG, Lynch M. Drift-barrier hypothesis and mutation-rate evolution. Proc Natl Acad Sci USA. 2012; 109 :18488–18492. [ PMC free article ] [ PubMed ] [ Google Scholar ]
- Teixeira L, Ferreira A, Ashburner M (2008) The bacterial symbiont Wolbachia induces resistance to RNA viral infections in Drosophila melanogaster . PLoS Biol 6:e2 [ PMC free article ] [ PubMed ]
- Timmis JN, Ayliffe MA, Huang CY, Martin W. Endosymbiotic gene transfer: organelle genomes forge eukaryotic chromosomes. Nat Rev Genet. 2004; 5 :123–135. [ PubMed ] [ Google Scholar ]
- Tin A, Grams ME, Ashar FN, Lane JA, Rosenberg AZ, Grove ML, et al. Association between mitochondrial DNA copy number in peripheral blood and incident CKD in the atherosclerosis risk in communities study. J Am Soc Nephrol. 2016; 27 :2467–2473. [ PMC free article ] [ PubMed ] [ Google Scholar ]
- Turelli M, Hoffmann AA (1991) Rapid spread of an inherited incompatibility factor in California Drosophila . Nature 353:440–442 [ PubMed ]
- Turelli M, Hoffmann AA, McKechnie SW. Dynamics of cytoplasmic incompatibility and mtDNA variation in natural Drosophila simulans populations. Genetics. 1992; 132 :713–723. [ PMC free article ] [ PubMed ] [ Google Scholar ]
- Utarini A, Indriani C, Ahmad RA, Tantowijoyo W, Arguni E, Ansari MR, et al. Efficacy of wolbachia-infected mosquito deployments for the control of dengue. N. Engl J Med. 2021; 384 :2177–2186. [ PMC free article ] [ PubMed ] [ Google Scholar ]
- Wagner DB, Dong J, Carlson MR, Yanchuk AD (1991) Paternal leakage of mitochondrial DNA in Pinus . Theor Appl Genet 82:510–514 [ PubMed ]
- Wallace DC. Mitochondrial DNA mutations and neuromuscular disease. Trends Genet. 1989; 5 :9–13. [ PubMed ] [ Google Scholar ]
- Waneka G, Svendsen JM, Havird JC, Sloan DB (2021) Mitochondrial mutations in Caenorhabditis elegans show signatures of oxidative damage and an AT-bias. Genetics 219:iyab116 [ PMC free article ] [ PubMed ]
- Waneka G, Vasquez YM, Bennett GM, Sloan DB (2021) Mutational pressure drives differential genome conservation in two bacterial endosymbionts of sap-feeding insects. Genome Biol Evol 13:evaa254 [ PMC free article ] [ PubMed ]
- Watanabe K, Yukuhiro F, Matsuura Y, Fukatsu T, Noda H. Intrasperm vertical symbiont transmission. Proc Natl Acad Sci USA. 2014; 111 :7433–7437. [ PMC free article ] [ PubMed ] [ Google Scholar ]
- Weihe A, Apitz J, Pohlheim F, Salinas-Hartwig A, Börner T (2009) Biparental inheritance of plastidial and mitochondrial DNA and hybrid variegation in Pelargonium . Mol Genet Genomics 282:587–593 [ PMC free article ] [ PubMed ]
- Weng M-L, Ruhlman TA, Jansen RK (2016) Plastid–nuclear interaction and accelerated coevolution in plastid ribosomal genes in Geraniaceae . Genome Biol Evol 8:1824–1838 [ PMC free article ] [ PubMed ]
- Wickett NJ, Fan Y, Lewis PO, Goffinet B (2008) Distribution and evolution of pseudogenes, gene losses, and a gene rearrangement in the plastid genome of the nonphotosynthetic liverwort, Aneura mirabilis ( Metzgeriales, Jungermanniopsida ). J Mol Evol 67:111–122 [ PubMed ]
- van Wijk KJ, Baginsky S. Plastid proteomics in higher plants: current state and future goals. Plant Physiol. 2011; 155 :1578–1588. [ PMC free article ] [ PubMed ] [ Google Scholar ]
- Williams AM, Friso G, van Wijk KJ, Sloan DB. Extreme variation in rates of evolution in the plastid Clp protease complex. Plant J. 2019; 98 :243–259. [ PubMed ] [ Google Scholar ]
- Wilton PR, Zaidi A, Makova K, Nielsen R. A population phylogenetic view of mitochondrial heteroplasmy. Genetics. 2018; 208 :1261–1274. [ PMC free article ] [ PubMed ] [ Google Scholar ]
- Wolfe KH, Li WH, Sharp PM. Rates of nucleotide substitution vary greatly among plant mitochondrial, chloroplast, and nuclear DNAs. Proc Natl Acad Sci USA. 1987; 84 :9054–9058. [ PMC free article ] [ PubMed ] [ Google Scholar ]
- Wolff JN, Nafisinia M, Sutovsky P, Ballard JWO. Paternal transmission of mitochondrial DNA as an integral part of mitochondrial inheritance in metapopulations of Drosophila simulans. Heredity. 2013; 110 :57–62. [ PMC free article ] [ PubMed ] [ Google Scholar ]
- Woloszynska M. Heteroplasmy and stoichiometric complexity of plant mitochondrial genomes—though this be madness, yet there’s method in’t. J Exp Bot. 2009; 61 :657–671. [ PubMed ] [ Google Scholar ]
- Wu ZQ, Liao XZ, Zhang XN, Tembrock LR, Broz A (2020) Genomic architectural variation of plant mitochondria—A review of multichromosomal structuring. J Syst Evol 60:160–168
- Wu Z, Sloan DB. Recombination and intraspecific polymorphism for the presence and absence of entire chromosomes in mitochondrial genomes. Heredity. 2019; 122 :647–659. [ PMC free article ] [ PubMed ] [ Google Scholar ]
- Wu Z, Waneka G, Broz AK, King CR, Sloan DB. MSH1 is required for maintenance of the low mutation rates in plant mitochondrial and plastid genomes. Proc Natl Acad Sci USA. 2020; 117 :16448–16455. [ PMC free article ] [ PubMed ] [ Google Scholar ]
- Xia H, Zhao W, Shi Y, Wang X-R, Wang B. Microhomologies are associated with tandem duplications and structural variation in plant mitochondrial genomes. Genome Biol Evol. 2020; 12 :1965–1974. [ PMC free article ] [ PubMed ] [ Google Scholar ]
- Yen JH, Barr AR (1971) New hypothesis of the cause of cytoplasmic incompatibility in Culex pipiens L. Nature 232:657–658 [ PubMed ]
- Zhang J, Ruhlman TA, Sabir J, Blazier JC, Jansen RK (2015) Coordinated rates of evolution between interacting plastid and nuclear genes in Geraniaceae . Plant Cell 27:563–573 [ PMC free article ] [ PubMed ]
- Zouros E (2000) The exceptional mitochondrial DNA system of the mussel family Mytilidae . Genes Genet Syst 75:313–318 [ PubMed ]
- Zouros E. Biparental inheritance through uniparental transmission: the doubly uniparental inheritance (DUI) of mitochondrial DNA. Evol Biol. 2013; 40 :1–31. [ Google Scholar ]
Extrachromosomal inheritance
Cite this chapter.
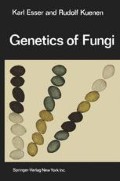
- Karl Esser 3 &
- Rudolf Kuenen 4
186 Accesses
As early as 1909 Carl Correns , one of the rediscoverers of the Mendelian laws, recognized that the nucleus did not have a monopoly on heredity. He was able to show that the cytoplasm also carries hereditary determinants . In the years following, many cases of extrachromosomal inheritance were described in plants and animals. It became clear that hereditary determinants occurring outside of the chromosomes have the capacity of self-replication and can be transmitted sexually or asexually. Nevertheless, in some cases the phenotypic manifestation of extrachromosomal hereditary determinants depends upon the genotype. The extrachromosomal determinants may reside in the plastids or other cytoplasmic components. While the term genome includes all of the chromosomal hereditary determinants, the extrachromosomal hereditary factors are subdivided into the plastome (factors in the plastids) and the Plasmone (factors in the remainder of the cytoplasm). Since the fungi lack plastids our concern here is with the plasmone.
- Neurospora Crassa
- Aspergillus Nidulans
- Respiratory Enzyme
- Infectious Particle
- Cytoplasmic Factor
These keywords were added by machine and not by the authors. This process is experimental and the keywords may be updated as the learning algorithm improves.
This is a preview of subscription content, log in via an institution to check access.
Access this chapter
- Available as PDF
- Read on any device
- Instant download
- Own it forever
- Compact, lightweight edition
- Dispatched in 3 to 5 business days
- Free shipping worldwide - see info
Tax calculation will be finalised at checkout
Purchases are for personal use only
Institutional subscriptions
Unable to display preview. Download preview PDF.
Arlett , C. F. : Induction of cytoplasmic mutations in Aspergillus nidulans . Nature (Lond.) 179 , 1250–1251 (1957).
Article CAS Google Scholar
— A system of cytoplasmic variation in Aspergillus nidulans . Heredity 15 , 377–388 (1960).
Article Google Scholar
— M. Grindle , and J. L. Jinks : The “red” cytoplasmic variant of Aspergillus nidulans . Heredity 17 , 197–209 (1962).
Article PubMed Google Scholar
Aschan , K. : Studies on dediploidisation mycelia of the basidiomycete Collybia velutipes . Svensk bot. T. 46 , 366–392 (1952).
Google Scholar
Aschan-Åberg , K. : Studies on dedikaryotization mycelia and of F 1 variants in Collybia velutipes . Svensk bot. T. 54 , 311–328 (1960).
Bautz , E. : Beeinflussung der Indophenolblaubildung (Nadi-Reaktion) in Hefezellen durch Röntgenstrahlen. Naturwissenschaften 41 , 375–376 (1954a).
— Untersuchungen über die Mitochondrien von Hefen. Ber. dtsch. bot. Ges. 67 , 281–288 (1954b).
— Mitochondrienfärbung mit Janusgrün bei Hefen. Naturwissenschaften 42 , 49–50 (1955).
—, u. H. Marquardt : Die Grana mit Mitochondrienfunktion in Hefezellen. Naturwissenschaften 40 , 531 (1953a).
— — Das Verhalten oxydierender Fermente in den Grana mit Mitochondrienfunktion der Hefezellen. Naturwissenschaften 40 , 531–532 (1953h).
— — Sprunghafte Änderungen des Verhaltens der Mitochondrien von Hefezellen gegenüber dem Nadi-Reagens. Naturwissenschaften 41 , 121–122 (1954).
Beale , G. H. : The genetics of Paramaecium aurelia. Cambridge (Engl.) 1954.
Beisson-Schecroun , J. : Incompatibilité cellulaire et interactions nucleoplasmiques dans les phénomènes de «barrage » chez le Podospora anserina. Ann. Génét. 4 , 4–50 (1962).
PubMed CAS Google Scholar
Bogen , H. J. , u. G. Kraepelin : Induktion atmungsdefekter Mutanten bei Saccharomyces cerevisiae mit asynchroner und synchronisierter Sprossung. Arch. Mikrobiol. 48 , 291–298 (1964).
Article PubMed CAS Google Scholar
Bulder , C. J. E. A. : Induction of petite mutation and inhibition of synthesis of respiratory enzymes in various yeasts. Antonie v. Leeuwenhoek 30 , 1–9 (1964).
Caspari , E. : Cytoplasmic inheritance. Advanc. Genet. 2 , 1–66 (1948).
Catcheside , D. G. : Introduction to a discussion on the cytoplasm in variation and development. Proc. roy. Soc. B 148 , 285–290 (1958).
Cytoplasmic inheritance. Nature (Lond.) 184 , 1012–1015 (1959).
Chevaugeon , J. , et S. Digbeu : Un second facteur cytoplasmique infectant chez Pestalozzia annulata. C. R. Acad. Sci. (Paris) 251 , 3043–3045 (1960).
CAS Google Scholar
—, et C. Lefort : Sur l’apparition régulière d’un «mutant» infectant chez un champignon du genre Pestalozzia. C. R. Acad. Sci. (Paris) 250 , 2247–2249 (1960).
Correns , C. : Zur Kenntnis der Rolle von Kern und Plasma bei der Vererbung. Z. indukt. Abstamm.- u. Vererb.-L. 2 , 331–340 (1909).
— Nichtmendelnde Vererbung. In: E. Bauer u. M. Hartmann (Hrsg.), Handbuch der Vererbungswissenschaft, Bd. II, S. 1–159. Berlin 1937.
Cuzin , F. : Apparition régulière chez Curvularia pallescenz , d’une variation sectorielle contagieuse, non transmissible par les thallospores. C. R. Acad. Sci. (Paris) 252 , 1656–1658 (1961).
Day , P. R. : A cytoplasmatically controlled abnormality of the tetrades of Copvinus lagopus. Heredity 13 , 81–87 (1959).
Delbrück , M. : In: Unités biologiques douées de continuité génétique: Colloques internat. du CNRS, vol. 7, p. 33, Paris 1949.
Dickson , H. : Observations of inheritance in Copvinus macrorhizus (Pers.) Rea. Ann. Bot. 50 , 719–733 (1936).
Dowding , E. S. , and A. Bakerspigel : Poor fruiters and barrage mutants in Gelasinospora. Canad. J. Bot. 34 , 231–240 (1956).
Ephrussi , B. : Quelques problèmes de la génétique des microorganismes. Arch. Klaus-Stift. Vererb.-Forsch. 26 , 403–425 (1951).
— The interplay of heredity and environment in the synthesis of respiratory enzymes in yeast. Harvey Lect. Series 46 , 45–67 (1952).
— Nucleo-cytoplasmic relations in microorganisms. Oxford (Engl.) 1953.
— Die Bestandteile des cytochrombildenden Systems der Hefe. Naturwissenschaften 43 , 505–511 (1956).
—, and H. Hottinguer: Cytoplasmic constituents of heredity. Cold Spr. Harb. Symp. quant. Biol. 16 , 75–85 (1951).
— — et A. M. Chimènes : Actions de Facriflavine sur les levures. I. La mutation «petite colonie». Ann. Inst. Pasteur 76 , 351–364 (1949a).
— — et H. Roman : Sur le comportement des mutants a déficience respiratoire de la levure dans les croisements. Caryologia Vol. Suppl. 1112–1113 (1954).
— — — Supressiveness: A new factor in the genetic determinism of the synthesis of respiratory enzymes in yeast. Proc. nat. Acad. Sci. (Wash.) 41 , 1065–1071 (1955).
— — et J. Tavlitzki : Action de Facriflavine sur les levures. II. Étude génétique du mutant «petite colonie». Ann. Inst. Pasteur 76 , 419–450 (1949b).
—, et P. P. Slonimski : La synthèse adaptive des cytochromes chez la levure de boulangerie. Biochim. biophys. Acta (Amst.) 6 , 256–267 (1950).
— — Yeast mitochondria, subcellular units involved in the synthesis of respiratory enzymes in yeast. Nature (Lond.) 176 , 1207–1209 (1955).
Esser , K. : Die Incompatibilitätsbeziehungen zwischen geographischen Rassen von Podospora anserina (Ces.) Rehm. II. Die Wirkungsweise der Incompatibilitätsgene. Z. Vererbungsl. 90 , 29–52 (1959).
Faulkner , B. M. , and C. F. Arlett : The “minute” cytoplasmic variant of Aspergillus nidulans. Heredity 19 , 63–73 (1964).
Fink , H. : Klassifizierung von Kulturhefen mit Hilfe des Cytochromspektrums. Hoppe-Seylers Z. physiol. Chem. 210 , 197–219 (1932).
—, u. E. Berwald : Über die Umwandlung des Cytochromspektrums in Bierhefen. Biochem. Z. 258 , 141–153 (1933).
Fitzgerald , P. H. : Genetic and epigenetic factors controlling female sterility in Neurospora crassa. Heredity 18 , 47–62 (1963).
Fries , N. , and K. Aschan : The physiological heterogeneity of the dikaryotic mycelium of Polyporus abietinus investigated with the aid of micrurgical technique. Svensk bot. T. 46 , 429–445 (1952).
Gowdridge , B. M. : Heterocaryons between strains of Neurospora crassa with different cytoplasms. Genetics 41 , 780–789 (1956).
Grenson , M. : A gene-induced cytoplasmic mutation in yeast. Proc. XI. intern. Congr. of Genetics, vol. 1, p. 202, The Hague 1963.
Grindle , M. : Nucleo-cytoplasmic interactions in the “red” cytoplasmic variant of Aspergillus nidulans. Heredity 19 , 75–95 (1964).
Hagemann , R. : Plasmatische Vererbung. Jena 1964.
— Extrachromosomale Vererbung. In: Bünning , E. et al. (Hrsg.): Fortschritte der Botanik, Springer: Berlin-Heidelberg-New York 1966, pp. 202–216.
Harder , R. : Zur Frage nach der Rolle von Kern und Protoplasma im Zellgeschehen und bei der Übertragung von Eigenschaften. Z. Bot. 19 , 337–407 (1927 a).
— Über mikrochirurgische Operationen an Hymenomyceten. Z. wiss. Mikr. 44 , 173–182 (1927b).
Hardesty , B. A. , and H. K. Mitchell : The accumulation of free fatty acids in poky, a maternal inherited mutant of Neurospora crassa. Arch. Biochem. 100 , 330–334 (1963).
Hartman , P. E. , and C. J. Liu : Comparative cytology of wild type Saccharomyces and a respirationally deficient mutant. J. Bact. 67 , 77–85 (1954).
Haskins , F. A. , A. Tissières , H. K. Mitchell , and M. B. Mitchell : Cytochromes and the succinic acid oxidase system of poky strains of Neurospora. J. biol. Chem. 200 , 819–826 (1953).
Jacob , F. , P. Schaeffer , and E. L. Wollman : Episomic elements in bacteria. Microbiol. Genetics, X. Symp., pp. 67–91, London 1960.
Jinks , J.L. : Somatic selection in fungi. Nature (Lond.) 174 , 409–410 (1954).
— Naturally occurring cytoplasmic changes in fungi. C. R. Lab. Carlsberg, Sér. Physiol. 26 , 183–203 (1956).
— Selection for cytoplasmic differences. Proc. roy. Soc. B 146 , 527–540 (1957).
— Cytoplasmic differentiation in fungi. Proc. roy. Soc. B 148 , 314–321 (1958).
Johnson , T. : Variation and the inheritance of certain characters in rust fungi. Cold Spr. Harb. Symp. quant. Biol. 11 , 85–93 (1946).
Joly , P. : Données récentes sur la génétique des champignons supérieurs (Ascomycètes et Basidiomycètes). Rev. Mycol. (Paris) 29 , 115–186 (1964).
Kimura , K. : On the diploidization by the double compatible diploid mycelium in the hymenomycetes. Bot. Mag. (Tokyo) 67 , 238–242 (1954).
Kraepelin , G. : Normalisierung des Atmungsdefektes bei Hefe. Rückführung stabilisierter RD -Mutanten in voll atmungsfähige Normalzellen. Arch. Mikrobiol. 48 , 299–305 (1964).
L’Héritier , P. : The CO 2 sensitivity problem in Drosophila. Cold Spr. Harb. Symp. quant. Biol. 16 , 99–112 (1951).
—Le problème de l’hérédité non chromosomique. Ann. Biol. 1 , 3–34 (1962).
Lindberg , G. D. : A transmissible disease of Helminthosporium victoriae. Phytopathology 49 , 29–32 (1959).
Lindegren , C. C. : Cytoplasmic inheritance. Ann. N.Y. Acad. Sci. 68 , 366–379 (1957).
Mahony , M. , and D. Wilkie : An instance of cytoplasmic inheritance in Aspergillus nidulans. Proc. roy. Soc. B 148 , 359–361 (1958).
— — Nucleo-cytoplasmic control of perithecial formation in Aspergillus nidulans. Proc. ryo. Soc. B 156 , 524–532 (1962).
Marcou , D. : Sur la longévité des souches de Podospora anserina cultivées à divers températures. C. R. Acad. Sci. (Paris) 239 , 895–897 (1954a).
— Sur le rajeunissement par le friod des souches de Podospora anserina. C. R. Acad. Sci. (Paris) 239 , 1153–1155 (1954b).
— Rajeunissement et arrêt de croissance chez Podospora anserina. C. R. Acad. Sci. (Paris) 244 , 661–663 (1957).
— Sur la déterminisme de la sénescence observée chez l’ascomycète Podospora anserina. Proc. X. intern. Congr. of Genetics, vol. II, p. 179, Montreal 1958.
— Notion de longévité et nature cytoplasmique de déterminent de la sénescence. Ann. Sci. nat. Bot. 2 , 653–764 (1961).
—, et J. Schecroun : La sénescence chez Podospora anserina pourrait être due à des particules cytoplasmiques infectantes. C. R. Acad. Sci. (Paris) 248 , 280–283 (1959).
Marquardt , H. : Die Natur der Erbträger im Zytoplasma. Ber. dtsch. bot. Ges. 65 , 198–217 (1952).
—, u. E. Bautz : Die Wirkung einiger Atmungsgifte auf das Verhalten von Hefe-Mitochondrien gegenüber der Nadi-Reaktion. Naturwissenschaften 41 , 361–362 (1954).
Mather , K. : Nucleus and cytoplasm in heredity and development. Proc. roy. Soc. B 148 , 362–369 (1958).
Michaelis , P. : Interactions between genes and cytoplasm in Epilobium. Cold Spr. Harb. Symp. quant. Biol. 16 , 121–129 (1951).
— Cytoplasmic inheritance in Epilobium and its theoretical significance. Advanc. Genet. 6 , 287–401 (1954).
— Genetical interactions between nucleus and cytoplasmic cell constituents. Path. et Biol. 9 , 769–772 (1961).
Mitchell , M. B. , and H. K. Mitchell : A case of ‘‘maternal’’ inheritance in Neurospora crassa. Proc. nat. Acad. Sci. (Wash.) 38 , 442–449 (1952).
— —, and A. Tissières : Mendelian and non-Mendelian factors affecting the cytochrome system in Neurospora crassa. Proc. nat. Acad. Sci. (Wash.) 39 , 606–613 (1953).
Muneta , J. , and A.M. Srb : Paternal transmission of extra-chromosomal properties in Neurospora. IX. Intern. Bot. Congr., vol. II, p. 274, Montreal (Canada) 1959.
Nanney , D. L. : The role of cytoplasm in heredity. In: W. D. McElroy and B. Glass (edits.), The chemical basis of heredity, pp. 134–166. Baltimore 1957.
Oehlkers , F. : Neue Überlegungen zum Problem der außerkaryotischen Vererbung. Z. indukt. Abstamm.- u. Vererb.-L. 84 , 213–250 (1952).
Oehlkers , F. — Außerkaryotische Vererbung. Naturwissenschaften 40 , 78–85 (1953).
Papazian , H. P. : Sectoring variants in Schizophyllum . Amer. J. Bot. 42 , 394–400 (1955).
— Sex and cytoplasm in the fungi. Trans. N.Y. Acad. Sci. 18 , 388–397 (1956).
— The genetics of basidiomycetes. Advanc. Genet. 9 , 41–69 (1958).
Quintanilha , A. , et S. Balle : Étude génétique des phénomènes de nanisme chez les hymenomycètes. Boll. Soc. Brot. 14 , 17–46 (1940).
Raut , C. : Cytochrome deficient yeast strains. Genetics 36 , 572 (1951).
— A cytochrome deficient mutant of S aecharomyces cerevisiae . Exp. Cell Res. 4 , 295–305 (1953).
— Heritable non-genic changes induced in yeast by ultraviolet light. J. cell. comp. Physiol. 44 , 463–475 (1954).
Renner , O. : Die pflanzlichen Piastiden als selbständige Elemente der genetischen Konstitution. Ber. sächs. Akad. Wiss. Leipzig, math.-physik. Kl. 86 , 241–266 (1934).
Rhoades , M. M. : Interaction of genie and non-genic hereditary units and the physiology of non-genic inheritance. In: W. Ruhland (Hrsg.), Handbuch der Pflanzenphysiologie, Bd. I, S. 19–57. Berlin-Göttingen-Heidelberg 1955.
Rizet , G. : Les phénomènes de barrage chez Podospora anserina . I. Analyse génétique des barrages entre souches S et s. Rev. Cytol. Biol, végét. 13 , 51–92 (1952).
— Sur la multiplicité des mécanismes génétiques conduisant à des barrages chez Podospora anserina . C. R. Acad. Sci. (Paris) 237 , 666–668 (1953a).
— Sur l’impossibilité d’obtenir la multiplication végétative interrompue et illimitée de l’ascomycète Podospora anserina . C. R. Acad. Sci. (Paris) 237 , 838–840 (1953b).
— Sur la longévité des souches de Podospora anserina . C. R. Acad. Sci. (Paris) 237 , 1106–1109 (1953 c).
— Les modifications qui conduisent à la sénescence chez Podospora sontelles de nature cytoplasmique ? C. R. Acad. Sci. (Paris) 244 , 663–665 (1957).
—, et D. Marcou : Longévité et sénescence chez l’acsomycète Podospora anserina . Compt. rend. VIII. Congr. intern. Bot. Sect., vol. 10, pp. 121–128, Paris 1954.
— — et J. Schecroun : Deux phénomènes d’héridité cytoplasmique chez l’ascomycète Podospora anserina . Bull. Soc. Franc. physiol. végét. 4 , 136–149 (1958).
—, et J. Schecroun : Sur les facteurs cytoplasmiques associés ou couple des gènes S— s chez Podospora anserina . C. R. Acad. Sci. (Paris) 249 , 2392–2394 (1959).
Roper , J. A. : Nucleo-cytoplasmic interactions in Aspergillus nidulans . Cold Spr. Harb. Symp. quant. Biol. 23 , 141–154 (1958).
Sager , R. , and F. J. Ryan : Cell heredity. New York and London 1961.
Schatz , G. , H. Tuppy u. J. Klima : Trennung und Charakterisierung cytoplasmatischer Partikel aus normaler und atmungsdefekter Bäckerhefe. Ein Beitrag zur Frage der genetischen Kontinuität der Mitochondrien von S aecharomyces cerevisiae . Z. Naturforsch. 18b , 145–153 (1963).
Schecroun , J. : Sur la réversion provoquée des souches s S en souches s chez Podospora anserina . C. R. Acad. Sci. (Paris) 246 , 1268–1270 (1958a).
— Sur la réversion provoquée d’une modification cytoplasmique chez Podospora anserina . Proc. X. intern. Congr. Genetics, vol. II, pp. 252–253, Montrale (Canada) 1958b.
— Sur la nature de la différence cytoplasmique entre souches s et s S de Podospora anserina . C. R. Acad. Sci. (Paris) 248 , 1394–1397 (1959).
Sharpe , S. : A closed system of cytoplasmic variation in Aspergillus glaucus . Proc. roy. Soc. B 148 , 355–359 (1958).
Sherman , F. : Respiration-deficient mutants of yeast. I. Genetics. Genetics 48 , 375–385 (1963).
— Mutants of yeast deficient in cytochrome C. Genetics 49 , 39–48 (1964).
Silagi , S. : Interactions between a cytoplasmic factor and nuclear genes in Neurospora crassa . Proc. XL intern. Congr. Genetics, vol. 1, pp. 202, The Hague 1963.
Slonimski , P. P. : Action de Facriflavine sur les levures. IV. Mode d’utilisation de glucose par les mutants «petite colonie». Ann. Inst. Pasteur 76 , 510–530 (1949a).
— Action de Facriflavine sur les levures. VIL Sur l’activité catalytique du cytochrome C des mutants «petite colonie» de la levure. Ann. Inst. Pasteur 77 , 774– 777 (1949b).
— Effet de l’oxygène sur la formation de quelques enzymes chez le mutant «petite colonie» de S aechar omyces cerevisiae . C. R. Acad. Sci. (Paris) 231 , 375–376 (1950).
— Recherches sur la formation des enzymes réspiratoires chez la levure. Thèse, Fac. des Sci. Paris 1952.
—, et B. Ephrussi : Action de l’acriflavines sur les levures. V. Le système de cytochromes des mutants «petite colonie». Ann. Inst. Pasteur 77 , 47–63 (1949).
— et H. M. Hirsch : Nouvelles données sur la constitution enzymatique du mutant «petite colonie» de S aechar omyces cerevisiae . C. R. Acad. Sci. (Paris) 235 , 741–743 (1952).
Sonneborn , T. M. : Kappa and related particles in Paramecium . Advanc. Virus Res. 6 , 231–356 (1959).
Srb , A. M. : Some consequences of nuclear cytoplasmic recombinations among various Neurosporas . Cold Spr. Harb. Symp. quant. Biol. 23 , 269–277 (1958).
Stubbe , W. : Genetische Analyse des Zusammenwirkens von Genom und Plasmon bei Oenothera . Z. Vererbungsl. 90 , 288–298 (1959).
— Extrem disharmonische Genom-Plastom-Kombinationen und väterliche Piastidenvererbung bei Oenothera . Z. Vererbungsl. 94 , 392–411 (1963).
Tavlitzki , J. : Action de Facriflavine sur les levures. III. Étude de la croissance des mutants «petite colonie». Ann. Inst. Pasteur 76 , 497–509 (1949).
Vandendries , R. : La tétrapolarité sexuelle de Pleurotus colombinus . Cellule 41 , 267–278 (1932).
Wettstein , F. v.: Die genetische und entwicklungsphysiologische Bedeutung des Cytoplasmas. Z. indukt. Abstamm.- u. Vererb.-L. 73 , 349–366 (1937).
Windisch , S. : Über Bildung und Bedeutung plasmatischer Mutanten von Kulturhefen. Brauerei, Wiss. Beil. 11 , 3–7 (1958).
Ycas , M. , and T. J. Starr : The effect of glycine and protoporphyrin on a cytochrome deficient yeast. J. Bact. 65 , 83–88 (1953).
Yotsuyanagi , Y. : Mitochondria and refractive granules in the yeast cells. Nature (Lond.) 176 , 1209 (1955).
— Études sur le chondriome de la levure. II. Chondriomes des mutants à déficience respiratoire. J. Ultrastruct. Res. 7 , 141–158 (1962).
References which have come to the authors’ attention after conclusion of the German manuscript A
Beale , G. H. : The role of the cytoplasm in heredity. Proc. roy. Soc. B 164 , 209–218 (1966).
Sager , R. : Mendelian and non-Mendelian heredity: a reappraisal. Proc. roy. Soc. B 164 , 290–297 (1966).
—, and Z. Ramanis : Recombination of nonchromosomal genes in Chlamydomonas . Proc. nat. Acad. Sci. (Wash.) 53 , 1053–1061 (1965).
Srb , A. M. : Extrachromosomal heredity in fungi. Reproduction: Molecular, subcellular and cellular, p. 191–211. New York 1965.
Avers , C. J. , and C. D. Dryfuss : Influence of added nucleosides on acriflavin induction of petite mutants in baker’s yeast. Nature (Lond.) 206 , 850 (1965).
— C. R. Pfeffer , and M. W. Rancourt : Acriflavine induction of different kinds of “petite” mitochondrial populations in Saccharornyces cerevisiae . J. Bact. 90 , 481–494 (1965).
Ephrussi , B. , et S. Grandchamp : Études sur la suppressivité des mutants à déficience respiratoire de la levure. I. Existence au niveau cellulaire de divers “degrés de suppressivité”. Heredity 20 , 1–7 (1965).
— H. Jakob et S. Grandchamp : Études sur la suppressivité des mutants à déficience respiratoire de la levure. II. Étapes de la mutation grande en petite provoquée par le facteur suppressif. Genetics 54 , 1–29 (1966).
Horn , P. , and D. Wilkie : Selective advantage of the cytoplasmic respiratory mutant of S aecharomyces cerevisiae in a cobalt medium. Heredity 21 , 625–635 (1967).
Jakob , H. : Complementation entre mutants à déficience respiratoire de Saccharomyces cerevisiae : Établissement et régulation de la respiration dans les zygotes et dans leur proche descendence. Genetics 52 , 75–98 (1965).
Kraepelin , G. : Zur Wirkung von 2,4-Dinitrophenol und Acridinorange auf Saccharomyces cerevisiae und dessen atmungsdefekte Mutante Mk. Arch. Mikrobiol. 50 , 52–58 (1965).
— Der Einfluß der Vorkultur auf die Induktion atmungsdefekter Hefemutanten durch Acridinorange. Arch. Mikrobiol. 50 , 59–62 (1965).
— Synchrone Vorgänge in asynchron sprossenden Saccharomyces- Kulturen: Mutationsauslösung und Abtötung durch Acridine. Arch. Mikrobiol. 50 , 63–67 (1965).
Morita , T. , and I. Mifuchi : Effect of methylene blue on the action of 4-nitroquinoline N-oxide and acriflavine in inducing respiration-deficient mutants of Saccharomyces cerevisiae . Jap. J. Microbiol. 9 , 123–129 (1965).
Moustacchi , E. , et H. Marcovich : Induction de la mutation «petite colonie» chez la levure par le 5-flurouracile. C. R. Acad. Sci. (Paris) 256 , 5646–5648 (1963).
—, and D. H. Williamson : Physiological variations in satellite components of yeast DNA detected by density gradient centrifugation. Biochem. biophys. Res. Commun. 23 , 56–61 (1966).
Sugimura , T. , K. Okabe , M. Nagao , and N. Gunge : A respiration-deficient mutant of Saccharomyces cerevisiae which accumulates porphyrins and lacks cytochromes. Biochim. biophys. Acta (Amst.) 115 , 267–275 (1966).
Tuppy , H. , E. Haslbrunner u. G. Schatz : Extranukleare Desoxyribonukleinsäure in aerob und anaerob gezüchteter normaler Bäckerhefe sowie in der atmungsdefizienten „petite“ -Mutante. Mh. Chem. 96 , 1831–1841 (1965).
Wintersberger , E. : Synthesis and function of mitochondrial ribonucleic acid. In: J. M. Tager, S. Papa, E. Quagliariello, and E. C. Slater: (eds.): Regulation of metabolic processes in mitochondria. Amsterdam 1966.
—, u. H. Tuppy : DNA-abhängige RNA-Synthese in isolierten Hefe-Mitochondrien. Biochem. Z. 341 , 399–408 (1965).
Heller , J. , and E. L. Smith : The amino acid sequence of cytochrome C of Neurospora crassa . Proc. nat. Acad. Sci. (Wash.) 54 , 1621–1625 (1965).
McDougall , K. J. , and T. H. Pittenger : A cytoplasmic variant of Neurospora crassa . Genetics 54 , 551–565 (1966).
Munkres , K. D. , and D. O. Woodward : On the genetics of enzyme locational specificity. Proc. nat. Acad. Sci. (Wash.) 55 , 1217–1224 (1966).
Silagi , S. : Interactions between an extrachromosomal factor, poky , and nuclear genes in Neurospora crassa . Genetics 52 , 341–347 (1965).
Srb , A. M. : Extrachromosomal factors in the genetic differentiation of Neurospora . Symp. Soc. Experim. Biol. XVII, Cell Different. 1963, p. 175–187.
Arlett , C. F. : The radiation sensitivity of a cytoplasmic mutant of Aspergillus nidulans . Int. J. Radiat. Biol. 10 , 539–549 (1966).
Chevaugeon , J. : Modification extra-chromosomique et âge du thalle chez le Pestalozzia annulata . C. R. Acad. Sci. (Paris) 255 , 1980–1982 (1962).
— Conditions de la différenciation du mycélium modifié chez le Pestalozzia annulata . C. R. Acad. Sci. (Paris) 255 , 3450–3452 (1962).
Chevaugeon , J. : Une période d’incubation s’interpose entre deux événe ments impliqués dans la modification extra-chromosomique du Pestalozzia annulata . C.R. Acad. Sci. (Paris) 257 , 217–220 (1963).
— Modification expérimentale du phénotype normal chez le Pestalozzia annulata . C. R. Acad. Sci. (Paris) 261 , 2730–2733 (1965).
— Mise en évidence de mécanismes de répression de la variation extrachromosomique chez le Pestalozzia annulata . C. R. Acad. Sci. (Paris) 263 , 120–123 (1966).
—, et L. Clouet : Temps et lieu des deux événements aléatoires impliqués dans la modification extra-chromosomique du Pestalozzia annulata . C. R. Acad. Sci. (Paris) 256 , 4068–4071 (1963).
— L. Clouet et G. Michel : Nutrition, croissance et modification extrachromosomique du Pestalozzia annulata . C. R. Acad. Sci. (Paris) 261 , 517–520 (1965).
Croft , J. H. : A reciprocal phenotypic instability affecting development in Aspergillus nidulans . Heredity 21 , 565–579 (1967).
Gregory , K. F. , and J. C. C. Huang : Tyrosinase inheritance in Streptomyces scabies . I. Genetic recombination. J. Bact. 87 , 1281–1286 (1964).
— — Tyrosinase inheritance in Streptomyces scabies . II. Induction of tyrosinase deficiency by acridine dyes. J. Bact. 87 , 1287–1294 (1964).
Kerr , S. : Disappearance of a genetic marker from a cytoplasmic hybrid Plasmodium of a true slime mold. Science 147 , 1586–1588 (1965).
Download references
Author information
Authors and affiliations.
Ruhr-Universität Bochum, Germany
Dr. phil. Karl Esser ( o. Professor )
Köln, Germany
Dr. rer. nat. Rudolf Kuenen ( Oberstudienrat )
You can also search for this author in PubMed Google Scholar
Rights and permissions
Reprints and permissions
Copyright information
© 1967 Springer-Verlag Berlin · Heidelberg
About this chapter
Esser, K., Kuenen, R. (1967). Extrachromosomal inheritance. In: Genetics of Fungi. Springer, Berlin, Heidelberg. https://doi.org/10.1007/978-3-642-86814-6_8
Download citation
DOI : https://doi.org/10.1007/978-3-642-86814-6_8
Publisher Name : Springer, Berlin, Heidelberg
Print ISBN : 978-3-642-86816-0
Online ISBN : 978-3-642-86814-6
eBook Packages : Springer Book Archive
Share this chapter
Anyone you share the following link with will be able to read this content:
Sorry, a shareable link is not currently available for this article.
Provided by the Springer Nature SharedIt content-sharing initiative
- Publish with us
Policies and ethics
- Find a journal
- Track your research
Work With Us- Join Our Team
Learn Genetics
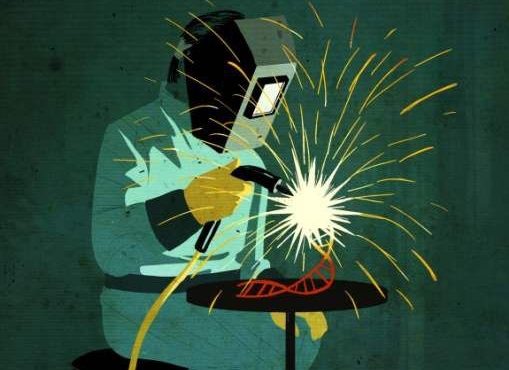
Extrachromosomal Inheritance- Definition, Criteria, Maternal Inheritance and Examples
“A non-mendelian pattern of inheritance governed by the DNA present in the cytoplasm is known as extrachromosomal inheritance or cytoplasmic inheritance.”
Our genetic material- DNA has arranged on chromosomes. It stores, transfer and express genetic information through replication, transcription and translation. Nuclear DNA- present in the nucleus of a cell, governs all phenotypes and is transmitted from parents to offspring in a specific pattern.
Autosomal dominant, autosomal recessive, X-linked dominant and X-linked recessive are several well-known patterns of inheritance. Genes present on autosomes follow autosomal inheritance whilst genes present on the X chromosome follow X-linked inheritance.
Broadly, when genotypes transfer from parents to offspring through reproduction are categorized in Mendelian inheritance. Nonetheless, several genes present in the cytoplasm don’t follow it. The process is known as cytoplasmic inheritance, non-Mendelian inheritance or extrachromosomal inheritance.
Organelle like chloroplast or mitochondria possesses cytoplasmic genes and these strings of DNA are often referred to as organelle DNAs .
In the present article, I am focusing on extrachromosomal inheritance, how it occurs and several examples. I will also explain maternal inheritance and the criteria of how it occurs. This present article will be a great learning opportunity for students regarding the present topic.
Let’s start with the basics,
Key Topics:
What is extrachromosomal inheritance?
The extrachromosomal inheritance also known as cytoplasmic inheritance or non-mendelian inheritance was first reported by Boris Ephrussi in yeast in 1949. As we said, organelles like chloroplast or mitochondria have their own genome. However, scientists still don’t understand how these organelles have created their own genomes .
One theory stated that it was a symbiotic relationship. It is believed that mitochondria were once free-living bacteria. Over a period of time, it has created a symbiotic relationship with eukaryotic cells and established itself in the cytoplasm. And later on, evolved as an organelle.
Similarly, the chloroplast in green plants comes from the free-living algae, established a symbiotic relationship with eukaryotic plant cells and settled into the cytoplasm of green plants. If you wish to learn about the present topic, we have given a link above in the article.
Both types of sub-genomes have well-developed DNA machinery. They can do their own replication, transcription and translation. In addition, the chloroplast has an antibiotic resistance gene which indicates that it has evolved from bacteria, previously.
The extrachromosomal genome comprises a few genes of several thousand base pairs, mostly are encoding rRNA, tRNA and other proteins for their DNA metabolism. Note that they have their own inheritance pattern.
Definition:
“The DNA present in the cytoplasm and not on chromosomes which follows the non-Mendelian pattern of inheritance is known as extrachromosomal inheritance.”
Now in the next section, I am going to explain about criteria, what are the criteria to categorize it as extrachromosomal DNA.
Criteria for extrachromosomal inheritance:
The extrachromosomal DNA follows a non-mendelian pattern of inheritance
Unlike the common nuclear DNA, genes present on organelle don’t follow the Mendelian style of Inheritance. It is a circular genome, lacking centromere and that’s why can’t segregate like the genomic DNA.
Their own machinery for protein synthesis
As these sub-genome has their own machinery for replication, transcription and translation, it synthesizes their own DNA and makes their own protein.
Maternal inheritance:
The extrachromosomal DNA is inherited from the maternal side.
The segregation is observed in somatic cells rather than germ cells, unlike nuclear inheritance.
Carl Correns in 1908, first reported non-mendelian inheritance in Mirabilis Jalapa plastid DNA. Another extrachromosomal inheritance was reported by M M. Rhoades in 1933. He postulated that the inheritance of male sterility in maize is governed by maternal inheritance and it becomes one of the greatest discoveries in science.
Another important point that makes extrachromosomal DNA even unique is maternal inheritance. Meaning, it transmits from mother to offspring. This shows that female individuals of the entire population are capable of transferring the cytoplasmic DNA.
No scientific evidence exists explaining why and how it occurs, however, One theory suggests that the female reproductive cell (ovum) is bigger than the sperm cell, contains more cytoplasm and more organelles than a male reproductive cell. Researchers expecting that this influences non-mendelian inheritance or maternal inheritance.
One of the classical examples of maternal inheritance is,
Cytoplasmic male sterility in maize.
In maize, nuclear genes do not play any significant role rather, the sterility is inherited through the egg cytoplasm in offspring.
When a male sterile plant is crossed with a normal fertile plant, all the F1 plants remain sterile. When all F1 sterile plants are backcross with a normal fertile plant, until all chromosomes from the male sterile line are exchanged to male fertile, the sterility persists in the progeny.
The image below makes it clear,
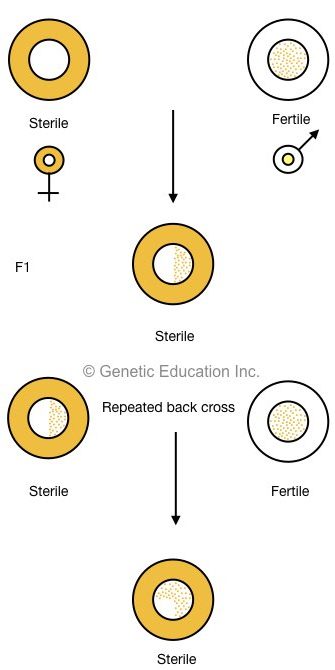
Generally, male-sterile lines are denoted as tcs, T (Texas), C (Cytoplasmic), S (Sterility). It was believed that T (Texas) cytoplasm is associated with susceptibility against several types of disease like leaf blight disease and yellow blight disease in maize.
Results indicating that chromosomal/nuclear DNA does not significantly affect male sterility (particularly in maize). Furthermore, most of the cytoplasm, carrying organelles are inherited from the maternal side. present explanation confirms that the sterility transmits from the cytoplasm.
Present findings become an important milestone in plant research and crop improvement. Maize corns can be uniformly developed in hybrid sterile maize plants.
Maternal inheritance is a miracle in nature, how some genotypes govern by the maternal side influences several important phenotypes. However, nuclear/chromosomal inheritance also occurs from the maternal side. Materna inheritance in complex, studies shows that several genotypes from the maternal side also influence phenotypes.
Read some of the interesting articles:
- The process of replication
The maternal-effect in snail:
The character of coiling in snail is governed by maternal inheritance. Snail- Limnaea peregra, has two types of shell coiling phenotypes: one is dextral that coil for the right side and another is sinistral that coils for the left side.
Here, the mother’s genotype (not a phenotype) is exclusively responsible for the development of coiling style. Assume that D + genotype codes for dextral (right side) coiling and D codes for sinistral coiling. The reciprocal cross of D + and D is shown in the figure:
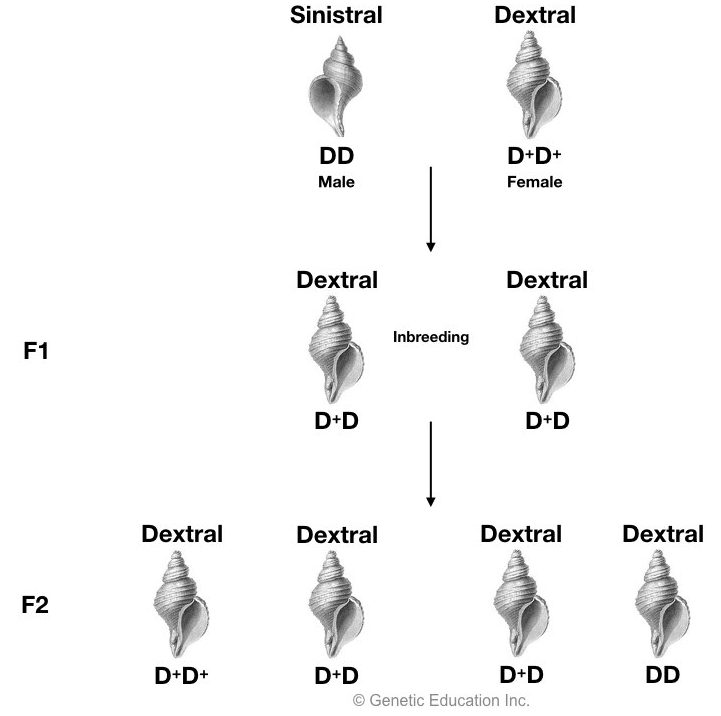
When crossing between D + D + female and DD male, all the F1 and F2 progeny become dextral as the mother is D + D + dextral Here, the DD recessive phenotype is not expressed and typical Mendelian 3:1 ratio is not obtained (all four are dextral).
In another condition when DD sinistral female is crossed with D + D + dextral male, F1 offspring become sinistral with genotype D + D, here mentioning genotype is important because the inheritance is governed by genotype not by phenotype.
When this F1 progeny is inbred (D + D * D + D) all the F2 progeny becomes dextral and coil for the right side. These results indicated that the phenotype of parents does not have any influence on the phenotype of progeny because although all of the F1 progeny are sinistral, all F2 offspring becomes dextral.
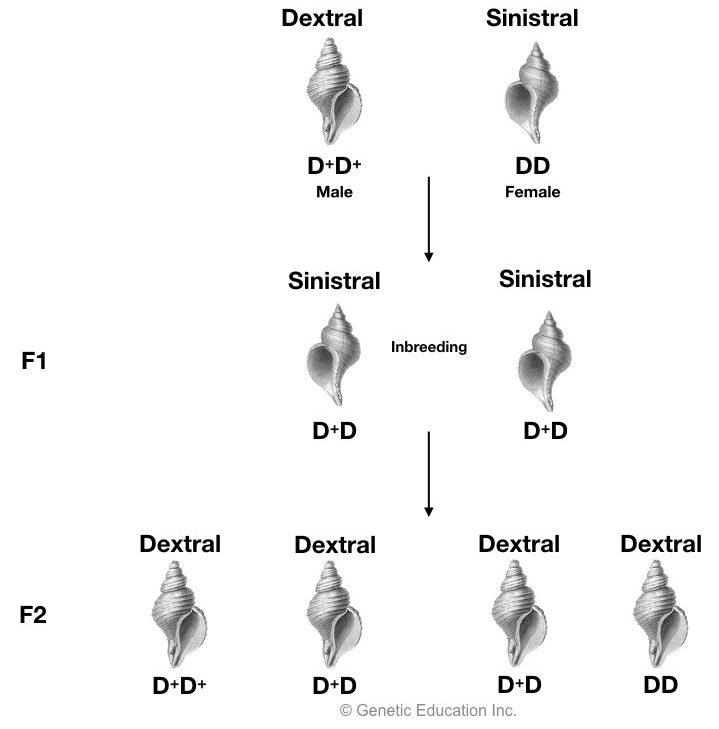
Detailed investigation shows that spindle formed during the second metaphase division decides the direction of coiling. The spindles of the dextral snail are tipped to the right and vice versa for sinistral. Interestingly, spindle arrangement in metaphase is controlled by maternal genes.
So the actual phenotype of “type of coiling” in snails is governed by maternal genes and it does not depend on the phenotype of any parents.
Interestingly in another scenario, the amount of cytoplasm transferred play a role in deciding the phenotype.
I nheritance of kappa particles in paramecium:
“Paramecin” is a substance found in some of the killer strains of paramecium which kill the sensitive strains. Paramecin production is governed by the kappa particles present in the cytoplasm of the paramecium.
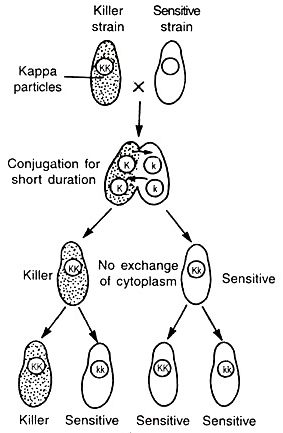
Here KK gene is responsible for the production of kappa particles which is dominant over the kk gene. In the case of inheritance of kappa particles, cytoplasmic exchange during conjugation plays a crucial role.
When KK killer strains are crossed with kk strains by conjugation, all the progeny obtained are heterozygous with genotype Kk but the phenotype of paramecium depends on the presence or absence of kappa particles and it will be influenced by the time of conjugation.
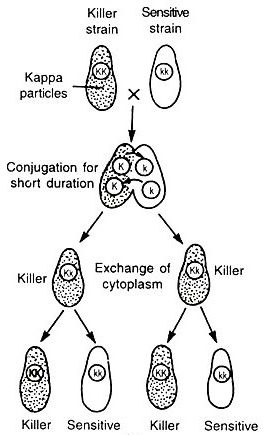
If both are conjugates for a shorter period of time, in the F1 generation killer strains remain killer and non-killer remain non-killer in the heterozygous condition. Here nuclear genes are only inherited and not cytoplasmic.
In another condition, if killer and non-killer strains are conjugated for a longer period of time, due to the exchange of kappa particles, sensitive strain receives kappa particles through cytoplasmic exchange and sensitive strains become killer in the F1 generation.
Extrachromosomal inheritance PDF, slide share and ppt:
Here I am enlisting some of the external resources that you can use for study. The PDFs, slide shares and ppts listed here are given from the trusted sources.
- Extrachromosomal inheritance pdf by Prof. Arif Ali
- Extrachromosomal inheritance pdf
Two best slide share and ppt options for extrachromosomal inheritance:
- Extrachromosomal inheritance Slideshare-1
- Extrachromosomal inheritance Slideshare-2
The cytoplasm is an important component of the cell, not only for transferring organelles but also for the inheritance of characters. Cytoplasmic inheritance and maternal inheritance are responsible for some of the disease condition in the human. Any defect in the inheritance of extrachromosomal genes results in serious physical, mental and biochemical problems.
The next section of this topic comprises information about the organelle DNAs. You can read it here: Extrachromosomal inheritance class 2: organelle DNA.
Article was written by- Tasneem Gandhi
The article reviewed by- Tushar Chauhan
This article is written by our dearest friend Tasneem Gandhi on the special request of team genetic education. We are heartily thankful to Tasneem.
Subscribe to our weekly newsletter for the latest blogs, articles and updates, and never miss the latest product or an exclusive offer.
Share this article
About The Author
Dr Tushar Chauhan
Related posts, chromosome- definition, structure, function and classification.
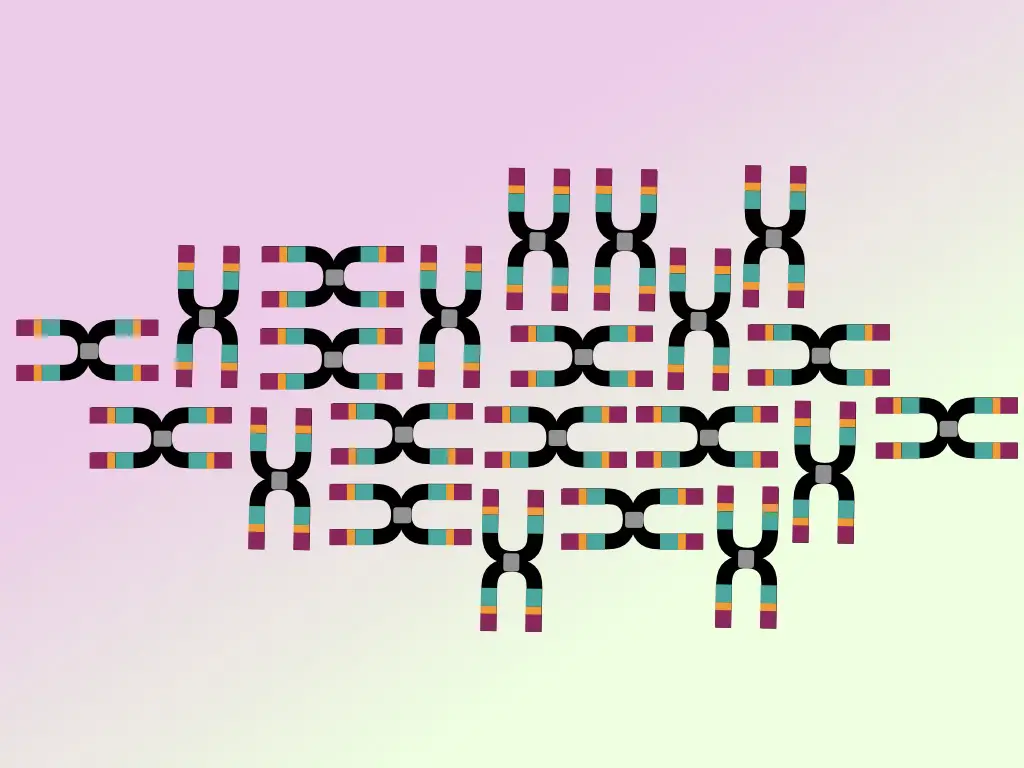
What is genome?- Definition, Structure and Function
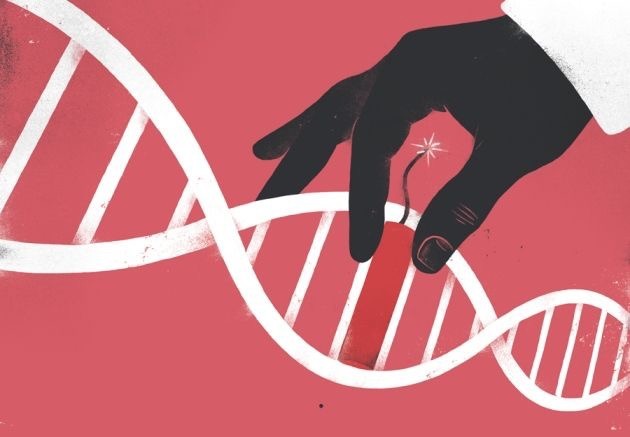
Download our free PCR worksheet now. Available for a limited period.
No thanks, I’m not interested!
Chapter 12: Development and Inheritance
Patterns of inheritance, learning objectives.
By the end of this section, you will be able to:
- Differentiate between genotype and phenotype
- Describe how alleles determine a person’s traits
- Summarize Mendel’s experiments and relate them to human genetics
- Explain the inheritance of autosomal dominant and recessive and sex-linked genetic disorders
We have discussed the events that lead to the development of a newborn. But what makes each newborn unique? The answer lies, of course, in the DNA in the sperm and oocyte that combined to produce that first diploid cell, the human zygote.
From Genotype to Phenotype
Each human body cell has a full complement of DNA stored in 23 pairs of chromosomes. The image below shows the pairs in a systematic arrangement called a karyotype . Among these is one pair of chromosomes, called the sex chromosomes , that determines the sex of the individual (XX in females, XY in males). The remaining 22 chromosome pairs are called autosomal chromosomes . Each of these chromosomes carries hundreds or even thousands of genes, each of which codes for the assembly of a particular protein—that is, genes are “expressed” as proteins. An individual’s complete genetic makeup is referred to as his or her genotype . The characteristics that the genes express, whether they are physical, behavioral, or biochemical, are a person’s phenotype .
You inherit one chromosome in each pair—a full complement of 23—from each parent. This occurs when the sperm and oocyte combine at the moment of your conception. Homologous chromosomes—those that make up a complementary pair—have genes for the same characteristics in the same location on the chromosome. Because one copy of a gene, an allele , is inherited from each parent, the alleles in these complementary pairs may vary. Take for example an allele that encodes for dimples. A child may inherit the allele encoding for dimples on the chromosome from the father and the allele that encodes for smooth skin (no dimples) on the chromosome from the mother.
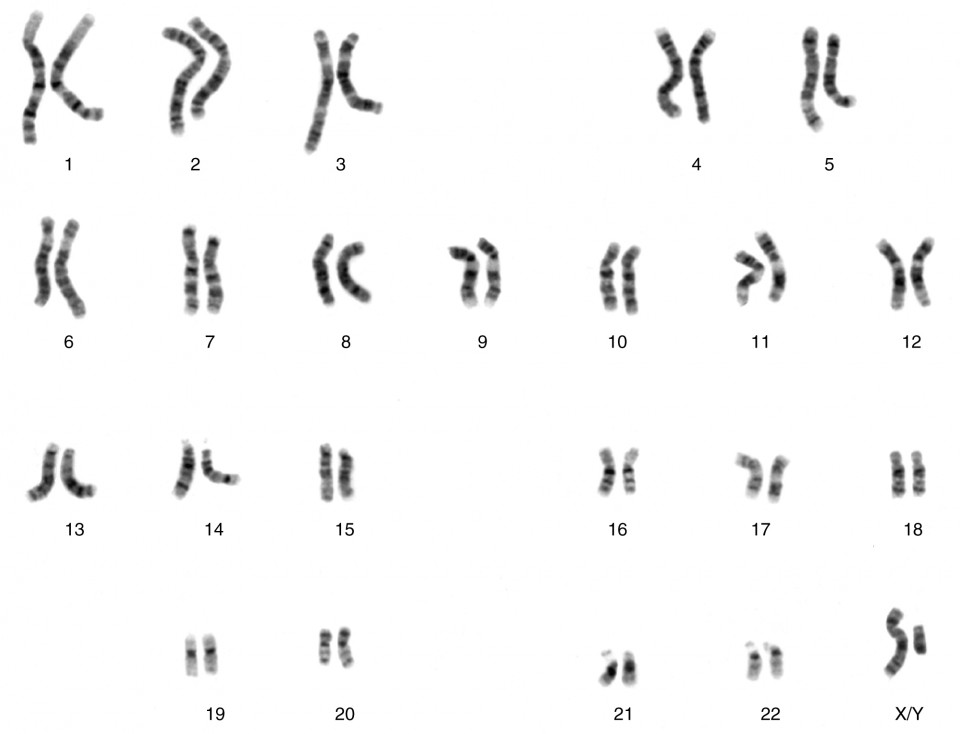
Figure 1. Each pair of chromosomes contains hundreds to thousands of genes. The banding patterns are nearly identical for the two chromosomes within each pair, indicating the same organization of genes. As is visible in this karyotype, the only exception to this is the XY sex chromosome pair in males. (credit: National Human Genome Research Institute)
Although a person can have two identical alleles for a single gene (a homozygous state), it is also possible for a person to have two different alleles (a heterozygous state). The two alleles can interact in several different ways. The expression of an allele can be dominant, for which the activity of this gene will mask the expression of a nondominant, or recessive, allele. Sometimes dominance is complete; at other times, it is incomplete. In some cases, both alleles are expressed at the same time in a form of expression known as codominance.
In the simplest scenario, a single pair of genes will determine a single heritable characteristic. However, it is quite common for multiple genes to interact to confer a feature. For instance, eight or more genes—each with their own alleles—determine eye color in humans. Moreover, although any one person can only have two alleles corresponding to a given gene, more than two alleles commonly exist in a population. This phenomenon is called multiple alleles. For example, there are three different alleles that encode ABO blood type; these are designated I A , I B , and i.
Over 100 years of theoretical and experimental genetics studies, and the more recent sequencing and annotation of the human genome, have helped scientists to develop a better understanding of how an individual’s genotype is expressed as their phenotype. This body of knowledge can help scientists and medical professionals to predict, or at least estimate, some of the features that an offspring will inherit by examining the genotypes or phenotypes of the parents. One important application of this knowledge is to identify an individual’s risk for certain heritable genetic disorders. However, most diseases have a multigenic pattern of inheritance and can also be affected by the environment, so examining the genotypes or phenotypes of a person’s parents will provide only limited information about the risk of inheriting a disease. Only for a handful of single-gene disorders can genetic testing allow clinicians to calculate the probability with which a child born to the two parents tested may inherit a specific disease.
Mendel’s Theory of Inheritance
Our contemporary understanding of genetics rests on the work of a nineteenth-century monk. Working in the mid-1800s, long before anyone knew about genes or chromosomes, Gregor Mendel discovered that garden peas transmit their physical characteristics to subsequent generations in a discrete and predictable fashion. When he mated, or crossed, two pure-breeding pea plants that differed by a certain characteristic, the first-generation offspring all looked like one of the parents. For instance, when he crossed tall and dwarf pure-breeding pea plants, all of the offspring were tall. Mendel called tallness dominant because it was expressed in offspring when it was present in a purebred parent. He called dwarfism recessive because it was masked in the offspring if one of the purebred parents possessed the dominant characteristic. Note that tallness and dwarfism are variations on the characteristic of height. Mendel called such a variation a trait . We now know that these traits are the expression of different alleles of the gene encoding height.
Mendel performed thousands of crosses in pea plants with differing traits for a variety of characteristics. And he repeatedly came up with the same results—among the traits he studied, one was always dominant, and the other was always recessive. (Remember, however, that this dominant–recessive relationship between alleles is not always the case; some alleles are codominant, and sometimes dominance is incomplete.)
Using his understanding of dominant and recessive traits, Mendel tested whether a recessive trait could be lost altogether in a pea lineage or whether it would resurface in a later generation. By crossing the second-generation offspring of purebred parents with each other, he showed that the latter was true: recessive traits reappeared in third-generation plants in a ratio of 3:1 (three offspring having the dominant trait and one having the recessive trait). Mendel then proposed that characteristics such as height were determined by heritable “factors” that were transmitted, one from each parent, and inherited in pairs by offspring.
In the language of genetics, Mendel’s theory applied to humans says that if an individual receives two dominant alleles, one from each parent, the individual’s phenotype will express the dominant trait. If an individual receives two recessive alleles, then the recessive trait will be expressed in the phenotype. Individuals who have two identical alleles for a given gene, whether dominant or recessive, are said to be homozygous for that gene (homo- = “same”). Conversely, an individual who has one dominant allele and one recessive allele is said to be heterozygous for that gene (hetero- = “different” or “other”). In this case, the dominant trait will be expressed, and the individual will be phenotypically identical to an individual who possesses two dominant alleles for the trait.
It is common practice in genetics to use capital and lowercase letters to represent dominant and recessive alleles. Using Mendel’s pea plants as an example, if a tall pea plant is homozygous, it will possess two tall alleles ( TT ). A dwarf pea plant must be homozygous because its dwarfism can only be expressed when two recessive alleles are present ( tt ). A heterozygous pea plant ( Tt ) would be tall and phenotypically indistinguishable from a tall homozygous pea plant because of the dominant tall allele. Mendel deduced that a 3:1 ratio of dominant to recessive would be produced by the random segregation of heritable factors (genes) when crossing two heterozygous pea plants. In other words, for any given gene, parents are equally likely to pass down either one of their alleles to their offspring in a haploid gamete, and the result will be expressed in a dominant–recessive pattern if both parents are heterozygous for the trait.
Because of the random segregation of gametes, the laws of chance and probability come into play when predicting the likelihood of a given phenotype. Consider a cross between an individual with two dominant alleles for a trait ( AA ) and an individual with two recessive alleles for the same trait ( aa ). All of the parental gametes from the dominant individual would be A , and all of the parental gametes from the recessive individual would be a . All of the offspring of that second generation, inheriting one allele from each parent, would have the genotype Aa , and the probability of expressing the phenotype of the dominant allele would be 4 out of 4, or 100 percent.
This seems simple enough, but the inheritance pattern gets interesting when the second-generation Aa individuals are crossed. In this generation, 50 percent of each parent’s gametes are A and the other 50 percent are a . By Mendel’s principle of random segregation, the possible combinations of gametes that the offspring can receive are AA , Aa , aA (which is the same as Aa ), and aa . Because segregation and fertilization are random, each offspring has a 25 percent chance of receiving any of these combinations. Therefore, if an Aa × Aa cross were performed 1000 times, approximately 250 (25 percent) of the offspring would be AA ; 500 (50 percent) would be Aa (that is, Aa plus aA ); and 250 (25 percent) would be aa . The genotypic ratio for this inheritance pattern is 1:2:1. However, we have already established that AA and Aa (and aA ) individuals all express the dominant trait (i.e., share the same phenotype), and can therefore be combined into one group. The result is Mendel’s third-generation phenotype ratio of 3:1.
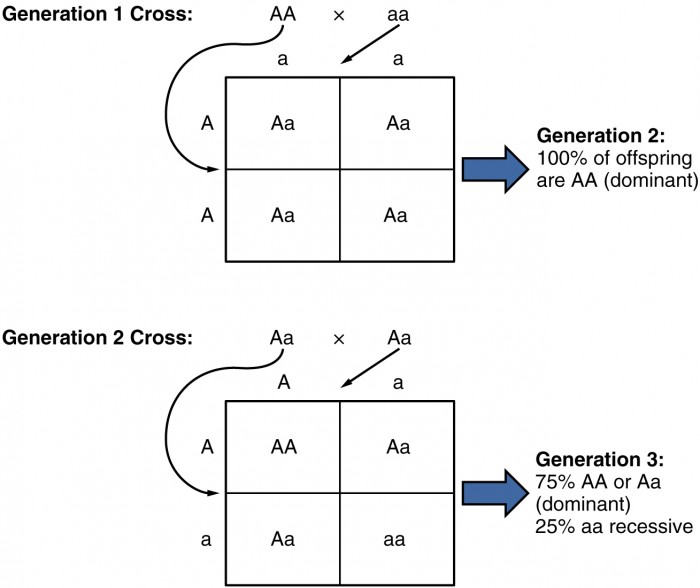
Figure 2. In the formation of gametes, it is equally likely that either one of a pair alleles from one parent will be passed on to the offspring. This figure follows the possible combinations of alleles through two generations following a first-generation cross of homozygous dominant and homozygous recessive parents. The recessive phenotype, which is masked in the second generation, has a 1 in 4, or 25 percent, chance of reappearing in the third generation.
Mendel’s observation of pea plants also included many crosses that involved multiple traits, which prompted him to formulate the principle of independent assortment. The law states that the members of one pair of genes (alleles) from a parent will sort independently from other pairs of genes during the formation of gametes. Applied to pea plants, that means that the alleles associated with the different traits of the plant, such as color, height, or seed type, will sort independently of one another. This holds true except when two alleles happen to be located close to one other on the same chromosome. Independent assortment provides for a great degree of diversity in offspring.
Mendelian genetics represent the fundamentals of inheritance, but there are two important qualifiers to consider when applying Mendel’s findings to inheritance studies in humans. First, as we’ve already noted, not all genes are inherited in a dominant–recessive pattern. Although all diploid individuals have two alleles for every gene, allele pairs may interact to create several types of inheritance patterns, including incomplete dominance and codominance.
Secondly, Mendel performed his studies using thousands of pea plants. He was able to identify a 3:1 phenotypic ratio in second-generation offspring because his large sample size overcame the influence of variability resulting from chance. In contrast, no human couple has ever had thousands of children. If we know that a man and woman are both heterozygous for a recessive genetic disorder, we would predict that one in every four of their children would be affected by the disease. In real life, however, the influence of chance could change that ratio significantly. For example, if a man and a woman are both heterozygous for cystic fibrosis, a recessive genetic disorder that is expressed only when the individual has two defective alleles, we would expect one in four of their children to have cystic fibrosis. However, it is entirely possible for them to have seven children, none of whom is affected, or for them to have two children, both of whom are affected. For each individual child, the presence or absence of a single gene disorder depends on which alleles that child inherits from his or her parents.
Autosomal Dominant Inheritance
In the case of cystic fibrosis, the disorder is recessive to the normal phenotype. However, a genetic abnormality may be dominant to the normal phenotype. When the dominant allele is located on one of the 22 pairs of autosomes (non-sex chromosomes), we refer to its inheritance pattern as autosomal dominant . An example of an autosomal dominant disorder is neurofibromatosis type I, a disease that induces tumor formation within the nervous system that leads to skin and skeletal deformities. Consider a couple in which one parent is heterozygous for this disorder (and who therefore has neurofibromatosis), Nn , and one parent is homozygous for the normal gene, nn . The heterozygous parent would have a 50 percent chance of passing the dominant allele for this disorder to his or her offspring, and the homozygous parent would always pass the normal allele. Therefore, four possible offspring genotypes are equally likely to occur: Nn , Nn , nn , and nn . That is, every child of this couple would have a 50 percent chance of inheriting neurofibromatosis. This inheritance pattern is shown in the table below, in a form called a Punnett square , named after its creator, the British geneticist Reginald Punnett.
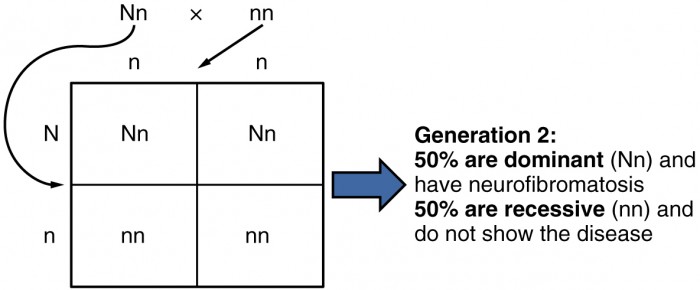
Figure 3. Inheritance pattern of an autosomal dominant disorder, such as neurofibromatosis, is shown in a Punnett square.
Other genetic diseases that are inherited in this pattern are achondroplastic dwarfism, Marfan syndrome, and Huntington’s disease. Because autosomal dominant disorders are expressed by the presence of just one gene, an individual with the disorder will know that he or she has at least one faulty gene. The expression of the disease may manifest later in life, after the childbearing years, which is the case in Huntington’s disease (discussed in more detail later in this section).
Autosomal Recessive Inheritance
When a genetic disorder is inherited in an autosomal recessive pattern, the disorder corresponds to the recessive phenotype. Heterozygous individuals will not display symptoms of this disorder, because their unaffected gene will compensate. Such an individual is called a carrier . Carriers for an autosomal recessive disorder may never know their genotype unless they have a child with the disorder.
An example of an autosomal recessive disorder is cystic fibrosis (CF), which we introduced earlier. CF is characterized by the chronic accumulation of a thick, tenacious mucus in the lungs and digestive tract. Decades ago, children with CF rarely lived to adulthood. With advances in medical technology, the average lifespan in developed countries has increased into middle adulthood. CF is a relatively common disorder that occurs in approximately 1 in 2000 Caucasians. A child born to two CF carriers would have a 25 percent chance of inheriting the disease. This is the same 3:1 dominant:recessive ratio that Mendel observed in his pea plants would apply here. The pattern is shown in the image below, using a diagram that tracks the likely incidence of an autosomal recessive disorder on the basis of parental genotypes.
On the other hand, a child born to a CF carrier and someone with two unaffected alleles would have a 0 percent probability of inheriting CF, but would have a 50 percent chance of being a carrier. Other examples of autosome recessive genetic illnesses include the blood disorder sickle-cell anemia, the fatal neurological disorder Tay–Sachs disease, and the metabolic disorder phenylketonuria.
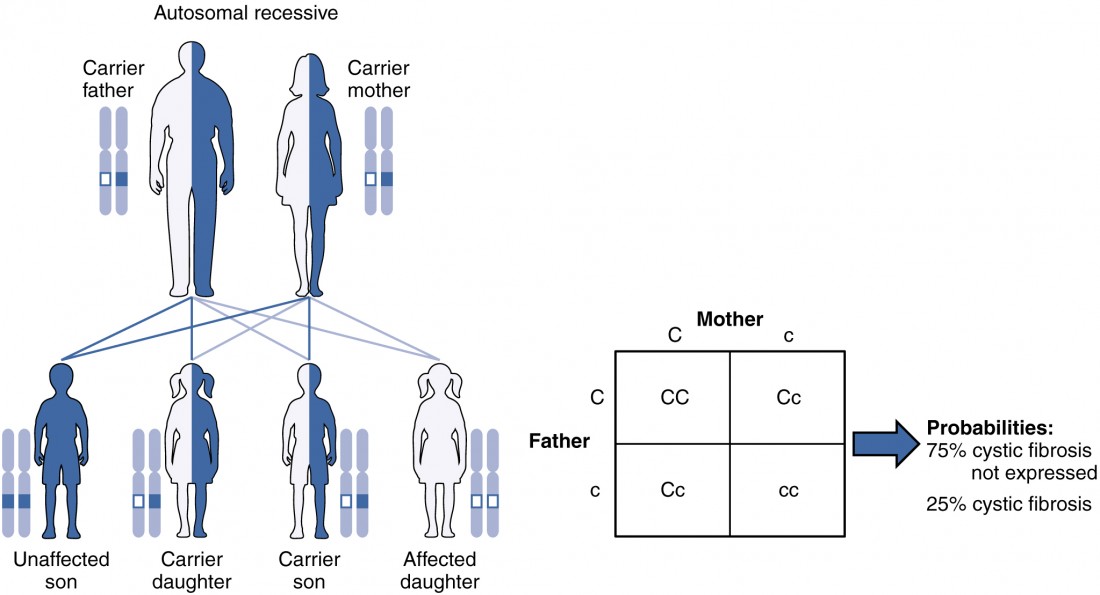
Figure 4. The inheritance pattern of an autosomal recessive disorder with two carrier parents reflects a 3:1 probability of expression among offspring. (credit: U.S. National Library of Medicine)
X-linked Dominant or Recessive Inheritance
An X-linked transmission pattern involves genes located on the X chromosome of the 23rd pair. Recall that a male has one X and one Y chromosome. When a father transmits a Y chromosome, the child is male, and when he transmits an X chromosome, the child is female. A mother can transmit only an X chromosome, as both her sex chromosomes are X chromosomes.
X-linked Dominant Inheritance
When an abnormal allele for a gene that occurs on the X chromosome is dominant over the normal allele, the pattern is described as X-linked dominant . This is the case with vitamin D–resistant rickets: an affected father would pass the disease gene to all of his daughters, but none of his sons, because he donates only the Y chromosome to his sons. If it is the mother who is affected, all of her children—male or female—would have a 50 percent chance of inheriting the disorder because she can only pass an X chromosome on to her children. For an affected female, the inheritance pattern would be identical to that of an autosomal dominant inheritance pattern in which one parent is heterozygous and the other is homozygous for the normal gene.
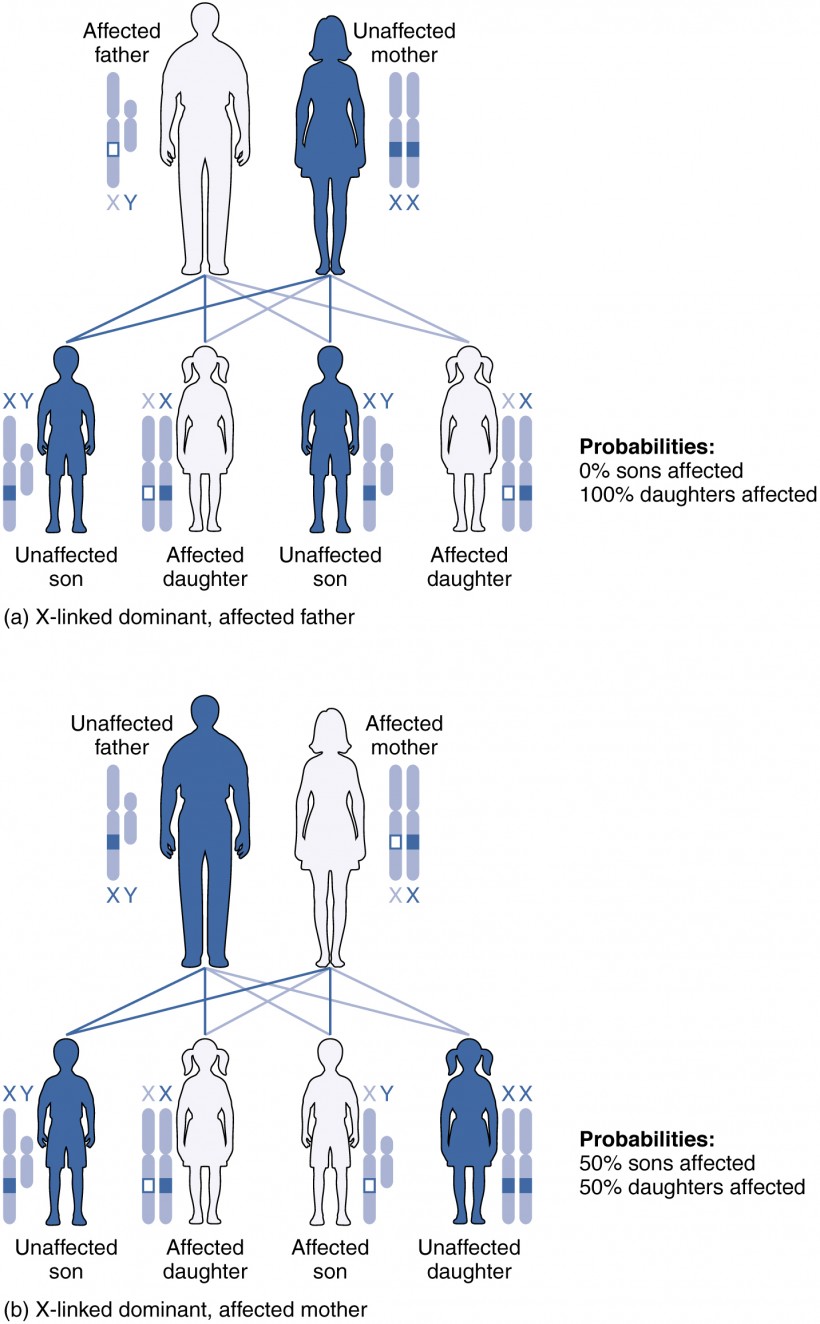
Figure 5. Click for a larger image. A chart of X-linked dominant inheritance patterns differs depending on whether (a) the father or (b) the mother is affected with the disease. (credit: U.S. National Library of Medicine)
X-linked Recessive Inheritance
X-linked recessive inheritance is much more common because females can be carriers of the disease yet still have a normal phenotype. Diseases transmitted by X-linked recessive inheritance include color blindness, the blood-clotting disorder hemophilia, and some forms of muscular dystrophy. For an example of X-linked recessive inheritance, consider parents in which the mother is an unaffected carrier and the father is normal. None of the daughters would have the disease because they receive a normal gene from their father. However, they have a 50 percent chance of receiving the disease gene from their mother and becoming a carrier. In contrast, 50 percent of the sons would be affected.
With X-linked recessive diseases, males either have the disease or are genotypically normal—they cannot be carriers. Females, however, can be genotypically normal, a carrier who is phenotypically normal, or affected with the disease. A daughter can inherit the gene for an X-linked recessive illness when her mother is a carrier or affected, or her father is affected. The daughter will be affected by the disease only if she inherits an X-linked recessive gene from both parents. As you can imagine, X-linked recessive disorders affect many more males than females. For example, color blindness affects at least 1 in 20 males, but only about 1 in 400 females.
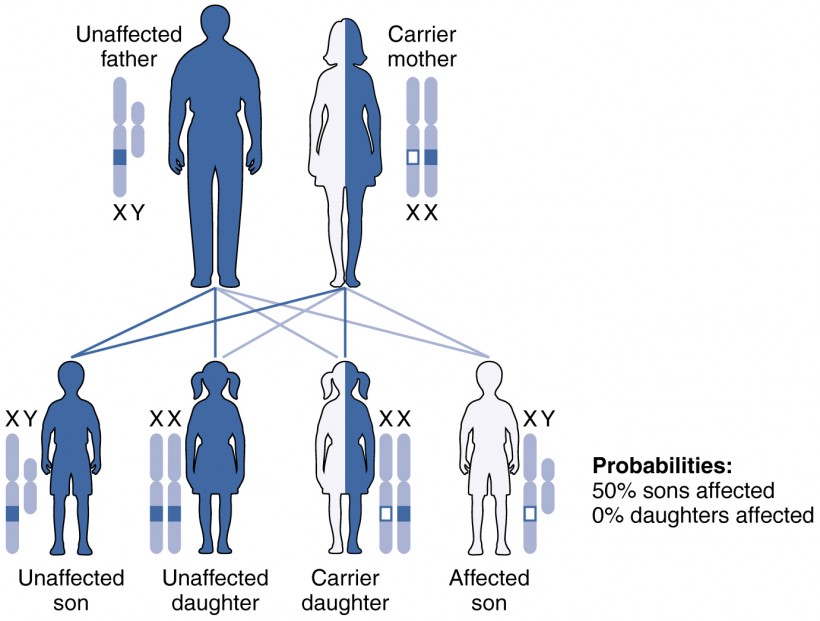
Figure 6. Given two parents in which the father is normal and the mother is a carrier of an X-linked recessive disorder, a son would have a 50 percent probability of being affected with the disorder, whereas daughters would either be carriers or entirely unaffected. (credit: U.S. National Library of Medicine)
Other Inheritance Patterns
Incomplete dominance.
Not all genetic disorders are inherited in a dominant–recessive pattern. In incomplete dominance , the offspring express a heterozygous phenotype that is intermediate between one parent’s homozygous dominant trait and the other parent’s homozygous recessive trait. An example of this can be seen in snapdragons when red-flowered plants and white-flowered plants are crossed to produce pink-flowered plants. In humans, incomplete dominance occurs with one of the genes for hair texture. When one parent passes a curly hair allele (the incompletely dominant allele) and the other parent passes a straight-hair allele, the effect on the offspring will be intermediate, resulting in hair that is wavy.
Codominance
Codominance is characterized by the equal, distinct, and simultaneous expression of both parents’ different alleles. This pattern differs from the intermediate, blended features seen in incomplete dominance. A classic example of codominance in humans is ABO blood type. People are blood type A if they have an allele for an enzyme that facilitates the production of surface antigen A on their erythrocytes. This allele is designated I A . In the same manner, people are blood type B if they express an enzyme for the production of surface antigen B. People who have alleles for both enzymes ( I A and I B ) produce both surface antigens A and B. As a result, they are blood type AB. Because the effect of both alleles (or enzymes) is observed, we say that the I A and I B alleles are codominant. There is also a third allele that determines blood type. This allele ( i ) produces a nonfunctional enzyme. People who have two i alleles do not produce either A or B surface antigens: they have type O blood. If a person has I A and i alleles, the person will have blood type A. Notice that it does not make any difference whether a person has two I A alleles or one I A and one i allele. In both cases, the person is blood type A. Because I A masks i , we say that I A is dominant to i . The following table summarizes the expression of blood type.
Lethal Alleles
Certain combinations of alleles can be lethal, meaning they prevent the individual from developing in utero, or cause a shortened life span. In recessive lethal inheritance patterns, a child who is born to two heterozygous (carrier) parents and who inherited the faulty allele from both would not survive. An example of this is Tay–Sachs, a fatal disorder of the nervous system. In this disorder, parents with one copy of the allele for the disorder are carriers. If they both transmit their abnormal allele, their offspring will develop the disease and will die in childhood, usually before age 5.
Dominant lethal inheritance patterns are much more rare because neither heterozygotes nor homozygotes survive. Of course, dominant lethal alleles that arise naturally through mutation and cause miscarriages or stillbirths are never transmitted to subsequent generations. However, some dominant lethal alleles, such as the allele for Huntington’s disease, cause a shortened life span but may not be identified until after the person reaches reproductive age and has children. Huntington’s disease causes irreversible nerve cell degeneration and death in 100 percent of affected individuals, but it may not be expressed until the individual reaches middle age. In this way, dominant lethal alleles can be maintained in the human population. Individuals with a family history of Huntington’s disease are typically offered genetic counseling, which can help them decide whether or not they wish to be tested for the faulty gene.
A mutation is a change in the sequence of DNA nucleotides that may or may not affect a person’s phenotype. Mutations can arise spontaneously from errors during DNA replication, or they can result from environmental insults such as radiation, certain viruses, or exposure to tobacco smoke or other toxic chemicals. Because genes encode for the assembly of proteins, a mutation in the nucleotide sequence of a gene can change amino acid sequence and, consequently, a protein’s structure and function. Spontaneous mutations occurring during meiosis are thought to account for many spontaneous abortions (miscarriages).
Chromosomal Disorders
Sometimes a genetic disease is not caused by a mutation in a gene, but by the presence of an incorrect number of chromosomes. For example, Down syndrome is caused by having three copies of chromosome 21. This is known as trisomy 21. The most common cause of trisomy 21 is chromosomal nondisjunction during meiosis. The frequency of nondisjunction events appears to increase with age, so the frequency of bearing a child with Down syndrome increases in women over 36. The age of the father matters less because nondisjunction is much less likely to occur in a sperm than in an egg.
Whereas Down syndrome is caused by having three copies of a chromosome, Turner syndrome is caused by having just one copy of the X chromosome. This is known as monosomy. The affected child is always female. Women with Turner syndrome are sterile because their sexual organs do not mature.
Career Connections: Genetic Counselor
Given the intricate orchestration of gene expression, cell migration, and cell differentiation during prenatal development, it is amazing that the vast majority of newborns are healthy and free of major birth defects. When a woman over 35 is pregnant or intends to become pregnant, or her partner is over 55, or if there is a family history of a genetic disorder, she and her partner may want to speak to a genetic counselor to discuss the likelihood that their child may be affected by a genetic or chromosomal disorder. A genetic counselor can interpret a couple’s family history and estimate the risks to their future offspring.
For many genetic diseases, a DNA test can determine whether a person is a carrier. For instance, carrier status for Fragile X, an X-linked disorder associated with mental retardation, or for cystic fibrosis can be determined with a simple blood draw to obtain DNA for testing. A genetic counselor can educate a couple about the implications of such a test and help them decide whether to undergo testing. For chromosomal disorders, the available testing options include a blood test, amniocentesis (in which amniotic fluid is tested), and chorionic villus sampling (in which tissue from the placenta is tested). Each of these has advantages and drawbacks. A genetic counselor can also help a couple cope with the news that either one or both partners is a carrier of a genetic illness, or that their unborn child has been diagnosed with a chromosomal disorder or other birth defect.
To become a genetic counselor, one needs to complete a 4-year undergraduate program and then obtain a Master of Science in Genetic Counseling from an accredited university. Board certification is attained after passing examinations by the American Board of Genetic Counseling. Genetic counselors are essential professionals in many branches of medicine, but there is a particular demand for preconception and prenatal genetic counselors.
Chapter Review
There are two aspects to a person’s genetic makeup. Their genotype refers to the genetic makeup of the chromosomes found in all their cells and the alleles that are passed down from their parents. Their phenotype is the expression of that genotype, based on the interaction of the paired alleles, as well as how environmental conditions affect that expression.
Working with pea plants, Mendel discovered that the factors that account for different traits in parents are discretely transmitted to offspring in pairs, one from each parent. He articulated the principles of random segregation and independent assortment to account for the inheritance patterns he observed. Mendel’s factors are genes, with differing variants being referred to as alleles and those alleles being dominant or recessive in expression. Each parent passes one allele for every gene on to offspring, and offspring are equally likely to inherit any combination of allele pairs. When Mendel crossed heterozygous individuals, he repeatedly found a 3:1 dominant–recessive ratio. He correctly postulated that the expression of the recessive trait was masked in heterozygotes but would resurface in their offspring in a predictable manner.
Human genetics focuses on identifying different alleles and understanding how they express themselves. Medical researchers are especially interested in the identification of inheritance patterns for genetic disorders, which provides the means to estimate the risk that a given couple’s offspring will inherit a genetic disease or disorder. Patterns of inheritance in humans include autosomal dominance and recessiveness, X-linked dominance and recessiveness, incomplete dominance, codominance, and lethality. A change in the nucleotide sequence of DNA, which may or may not manifest in a phenotype, is called a mutation.
Answer the question(s) below to see how well you understand the topics covered in the previous section.
Critical Thinking Questions
- Explain why it was essential that Mendel perform his crosses using a large sample size?
- How can a female carrier of an X-linked recessive disorder have a daughter who is affected?
- By using large sample sizes, Mendel minimized the effect of random variability resulting from chance. This allowed him to identify true ratios corresponding to dominant–recessive inheritance.
- The only way an affected daughter could be born is if the female carrier mated with a male who was affected. In this case, 50 percent of the daughters would be affected. Alternatively, but exceedingly unlikely, the daughter could become affected by a spontaneous mutation.
allele: alternative forms of a gene that occupy a specific locus on a specific gene
autosomal chromosome: in humans, the 22 pairs of chromosomes that are not the sex chromosomes (XX or XY)
autosomal dominant: pattern of dominant inheritance that corresponds to a gene on one of the 22 autosomal chromosomes
autosomal recessive: pattern of recessive inheritance that corresponds to a gene on one of the 22 autosomal chromosomes
carrier: heterozygous individual who does not display symptoms of a recessive genetic disorder but can transmit the disorder to his or her offspring
codominance: pattern of inheritance that corresponds to the equal, distinct, and simultaneous expression of two different alleles
dominant: describes a trait that is expressed both in homozygous and heterozygous form
dominant lethal: inheritance pattern in which individuals with one or two copies of a lethal allele do not survive in utero or have a shortened life span
genotype: complete genetic makeup of an individual
heterozygous: having two different alleles for a given gene
homozygous: having two identical alleles for a given gene
incomplete dominance: pattern of inheritance in which a heterozygous genotype expresses a phenotype intermediate between dominant and recessive phenotypes
karyotype: systematic arrangement of images of chromosomes into homologous pairs
mutation: change in the nucleotide sequence of DNA
phenotype: physical or biochemical manifestation of the genotype; expression of the alleles
Punnett square: grid used to display all possible combinations of alleles transmitted by parents to offspring and predict the mathematical probability of offspring inheriting a given genotype
recessive: describes a trait that is only expressed in homozygous form and is masked in heterozygous form
recessive lethal: inheritance pattern in which individuals with two copies of a lethal allele do not survive in utero or have a shortened life span
sex chromosomes: pair of chromosomes involved in sex determination; in males, the XY chromosomes; in females, the XX chromosomes
trait: variation of an expressed characteristic
X-linked: pattern of inheritance in which an allele is carried on the X chromosome of the 23rd pair
X-linked dominant: pattern of dominant inheritance that corresponds to a gene on the X chromosome of the 23rd pair
X-linked recessive: pattern of recessive inheritance that corresponds to a gene on the X chromosome of the 23rd pair
- Anatomy & Physiology. Provided by : OpenStax CNX. Located at : http://cnx.org/contents/[email protected] . License : CC BY: Attribution . License Terms : Download for free at http://cnx.org/contents/[email protected]
- Biology Article
- Chromosomal Theory Of Inheritance
Chromosomal Theory of Inheritance
What are chromosomes.
Chromosomes are a thread-like structure of nucleic acids and protein located within the nucleus of the living cells and are mainly involved in carrying genetic information in the form of genes.
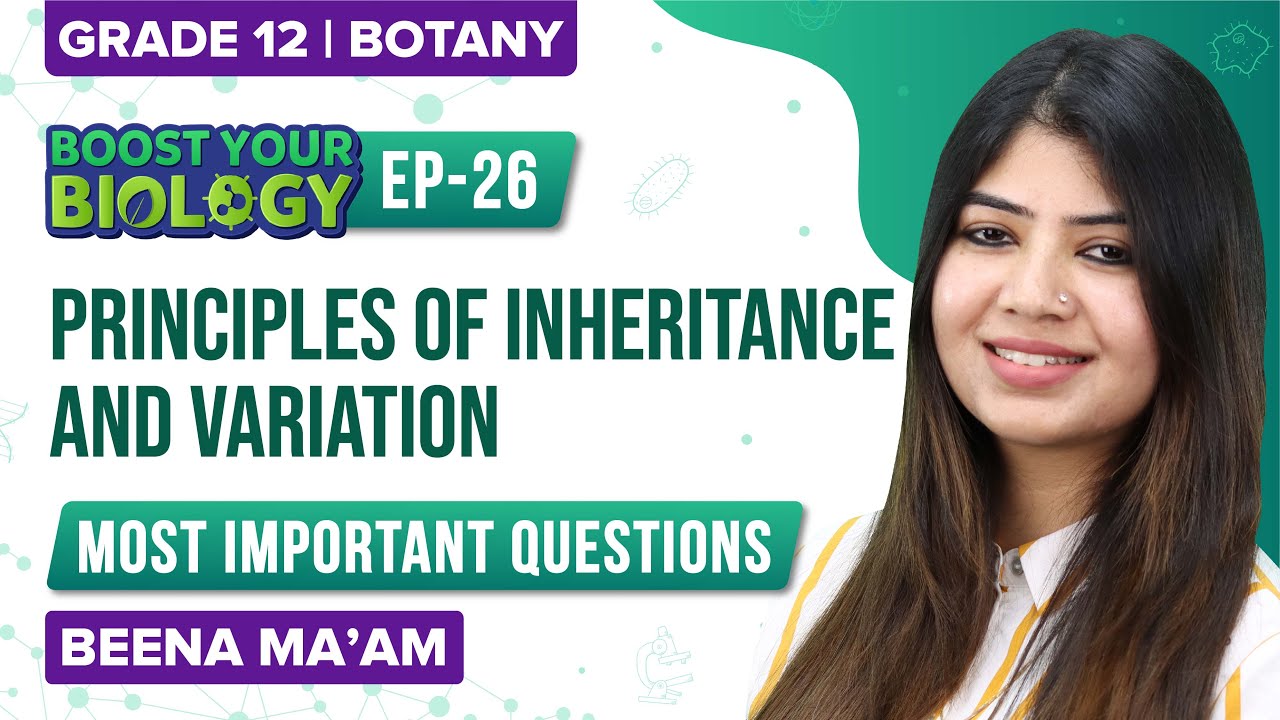
The Chromosomal Theory of Inheritance
The chromosomal theory of inheritance was given by Boveri and Sutton in the early 1900s. It is the fundamental theory of genetics. According to this theory, genes are the units of heredity and are found in the chromosomes.
Chromosomal Theory of Inheritance came into existence long after Mendelian genetics. During Mendel’s experimentation, the society was not acceptable to such drastic changes in their scientific ideas. They could not believe the existence of such discrete factors such as genes which would segregate without mixing as this did not support their idea of the constant changes leading to evolution. Moreover, the means of communication was poor at that time as a result of which, the information could not be conveyed to the masses. Also, Mendel’s mathematical approach to prove biological laws was unacceptable.
As time passed, scientists Vries, Correns and Tschermak discovered chromosomes which existed inside the nucleus. Sutton and Boveri discovered observed the behaviour of the chromosomes when the cells were divided. With the advancements in the microscope, this task became easier. Hence, they proved Mendel’s laws with the help of chromosomal movement. They showed the segregation of the chromosomes during the Anaphase of cell division. The idea of chromosomal segregation combined with the Mendelian principles gave rise to the chromosomal theory of Inheritance. The work was further carried forward and proved by T.H. Morgan, who used Drosophila melanogaster to show how sexual reproduction gave rise to variations.
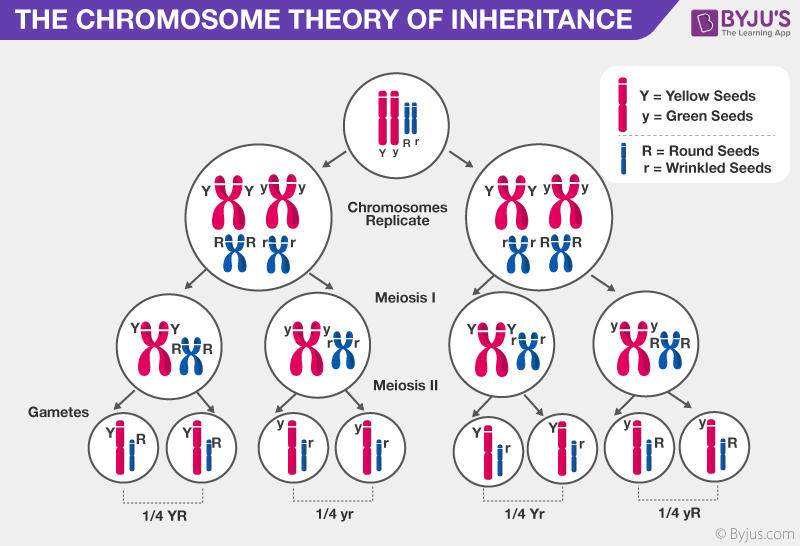
Chromosomal theory: Linkage and Genetic Recombination
Morgan observed that while crossing a set of characteristics, two genes did not segregate as per Mendel’s law. If two genes were present on the same chromosome , the probability of getting a parental combination was much higher in the next generation compared to the non-parental combination. This physical association of genes was termed as linkage. The term genetic recombination described the non-parental gene combinations in a dihybrid cross. Once, linked genes were discovered, the frequency of linked genes also influenced the appearance of traits in the next generation. A student of Morgan, Sturtevant discovered the position of linked genes on a chromosome by calculating their frequency of genetic recombination by the process of gene mapping. This method of generating a link map was extensively used during the Human Genome Project.
Also, read Human Genome Project
Observations of Chromosomal Theory of Inheritance
The Chromosomal Theory of Inheritance supports Mendel’s laws. Listed below are the observations of this theory:
- During the process of cell division-meiosis, the pairs of homologous chromosome move as discrete structures, which are independent of other pairs of chromosomes.
- There is a random distribution of chromosomes into the pre-gametes from each homologous pair.
- Each parent synthesizes gametes, which constitute only half of their chromosomal complement.
- Even though female (egg) and male (sperm) gametes differ in their size and morphology, they have the same number of chromosomes, submitting equal genetic contributions from each parent.
- The gametic chromosomes fuse during fertilization to produce offspring with the same number of a chromosome as their parents.
Some Important Questions on Chromosomal Theory of Inheritance
- Who discovered chromosomes?
Carl Wilhelm von Nageli , a Swiss botanist discovered chromosomes and he was a first person to study about the cell divisions
- What is the chromosomal theory of inheritance?
It is the fundamental theory of genetics which recognizes chromosomes as the carriers of genetic material.
- Who proposed the chromosomal theory of inheritance?
Theodor Boveri and Walter Sutton are the two scientists who were credited with developing the Chromosomal Theory of Inheritance.
- What is Mutations?
The mutation is defined as the change or the permanent alteration of the nucleotide sequence of the genome of an organism.
Stay tuned with BYJU’S to know more in detail about the chromosomal theory of inheritance.
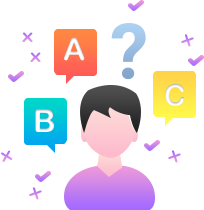
Put your understanding of this concept to test by answering a few MCQs. Click ‘Start Quiz’ to begin!
Select the correct answer and click on the “Finish” button Check your score and answers at the end of the quiz
Visit BYJU’S for all Biology related queries and study materials
Your result is as below
Request OTP on Voice Call
Leave a Comment Cancel reply
Your Mobile number and Email id will not be published. Required fields are marked *
Post My Comment

- Share Share
Register with BYJU'S & Download Free PDFs
Register with byju's & watch live videos.

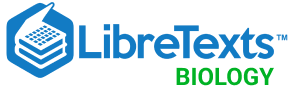
- school Campus Bookshelves
- menu_book Bookshelves
- perm_media Learning Objects
- login Login
- how_to_reg Request Instructor Account
- hub Instructor Commons
- Download Page (PDF)
- Download Full Book (PDF)
- Periodic Table
- Physics Constants
- Scientific Calculator
- Reference & Cite
- Tools expand_more
- Readability
selected template will load here
This action is not available.
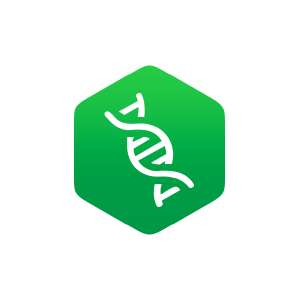
12.2: Chromosomal Basis of Inherited Disorders
- Last updated
- Save as PDF
- Page ID 76338
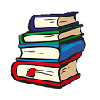
Skills to Develop
- Describe how a karyogram is created
- Explain how nondisjunction leads to disorders in chromosome number
- Compare disorders caused by aneuploidy
- Describe how errors in chromosome structure occur through inversions and translocations
Inherited disorders can arise when chromosomes behave abnormally during meiosis. Chromosome disorders can be divided into two categories: abnormalities in chromosome number and chromosomal structural rearrangements. Because even small segments of chromosomes can span many genes, chromosomal disorders are characteristically dramatic and often fatal.
Identification of Chromosomes
The isolation and microscopic observation of chromosomes forms the basis of cytogenetics and is the primary method by which clinicians detect chromosomal abnormalities in humans. A karyotype is the number and appearance of chromosomes, and includes their length, banding pattern, and centromere position. To obtain a view of an individual’s karyotype, cytologists photograph the chromosomes and then cut and paste each chromosome into a chart, or karyogram , also known as an ideogram (Figure \(\PageIndex{1}\)).
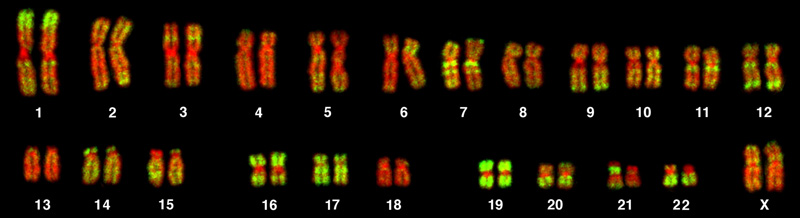
In a given species, chromosomes can be identified by their number, size, centromere position, and banding pattern. In a human karyotype, autosomes or “body chromosomes” (all of the non–sex chromosomes) are generally organized in approximate order of size from largest (chromosome 1) to smallest (chromosome 22). The X and Y chromosomes are not autosomes. However, chromosome 21 is actually shorter than chromosome 22. This was discovered after the naming of Down syndrome as trisomy 21, reflecting how this disease results from possessing one extra chromosome 21 (three total). Not wanting to change the name of this important disease, chromosome 21 retained its numbering, despite describing the shortest set of chromosomes. The chromosome “arms” projecting from either end of the centromere may be designated as short or long, depending on their relative lengths. The short arm is abbreviated p (for “petite”), whereas the long arm is abbreviated q (because it follows “p” alphabetically). Each arm is further subdivided and denoted by a number. Using this naming system, locations on chromosomes can be described consistently in the scientific literature.
Career Connection: Geneticists Use Karyograms to Identify Chromosomal Aberrations
Although Mendel is referred to as the “father of modern genetics,” he performed his experiments with none of the tools that the geneticists of today routinely employ. One such powerful cytological technique is karyotyping, a method in which traits characterized by chromosomal abnormalities can be identified from a single cell. To observe an individual’s karyotype, a person’s cells (like white blood cells) are first collected from a blood sample or other tissue. In the laboratory, the isolated cells are stimulated to begin actively dividing. A chemical called colchicine is then applied to cells to arrest condensed chromosomes in metaphase. Cells are then made to swell using a hypotonic solution so the chromosomes spread apart. Finally, the sample is preserved in a fixative and applied to a slide.
The geneticist then stains chromosomes with one of several dyes to better visualize the distinct and reproducible banding patterns of each chromosome pair. Following staining, the chromosomes are viewed using bright-field microscopy. A common stain choice is the Giemsa stain. Giemsa staining results in approximately 400–800 bands (of tightly coiled DNA and condensed proteins) arranged along all of the 23 chromosome pairs; an experienced geneticist can identify each band. In addition to the banding patterns, chromosomes are further identified on the basis of size and centromere location. To obtain the classic depiction of the karyotype in which homologous pairs of chromosomes are aligned in numerical order from longest to shortest, the geneticist obtains a digital image, identifies each chromosome, and manually arranges the chromosomes into this pattern (Figure \(\PageIndex{1}\)).
At its most basic, the karyogram may reveal genetic abnormalities in which an individual has too many or too few chromosomes per cell. Examples of this are Down Syndrome, which is identified by a third copy of chromosome 21, and Turner Syndrome, which is characterized by the presence of only one X chromosome in women instead of the normal two. Geneticists can also identify large deletions or insertions of DNA. For instance, Jacobsen Syndrome—which involves distinctive facial features as well as heart and bleeding defects—is identified by a deletion on chromosome 11. Finally, the karyotype can pinpoint translocations, which occur when a segment of genetic material breaks from one chromosome and reattaches to another chromosome or to a different part of the same chromosome. Translocations are implicated in certain cancers, including chronic myelogenous leukemia.
During Mendel’s lifetime, inheritance was an abstract concept that could only be inferred by performing crosses and observing the traits expressed by offspring. By observing a karyogram, today’s geneticists can actually visualize the chromosomal composition of an individual to confirm or predict genetic abnormalities in offspring, even before birth.
Disorders in Chromosome Number
Of all of the chromosomal disorders, abnormalities in chromosome number are the most obviously identifiable from a karyogram. Disorders of chromosome number include the duplication or loss of entire chromosomes, as well as changes in the number of complete sets of chromosomes. They are caused by nondisjunction , which occurs when pairs of homologous chromosomes or sister chromatids fail to separate during meiosis. Misaligned or incomplete synapsis, or a dysfunction of the spindle apparatus that facilitates chromosome migration, can cause nondisjunction. The risk of nondisjunction occurring increases with the age of the parents.
Nondisjunction can occur during either meiosis I or II, with differing results (Figure \(\PageIndex{2}\)). If homologous chromosomes fail to separate during meiosis I, the result is two gametes that lack that particular chromosome and two gametes with two copies of the chromosome. If sister chromatids fail to separate during meiosis II, the result is one gamete that lacks that chromosome, two normal gametes with one copy of the chromosome, and one gamete with two copies of the chromosome.
Art Connection
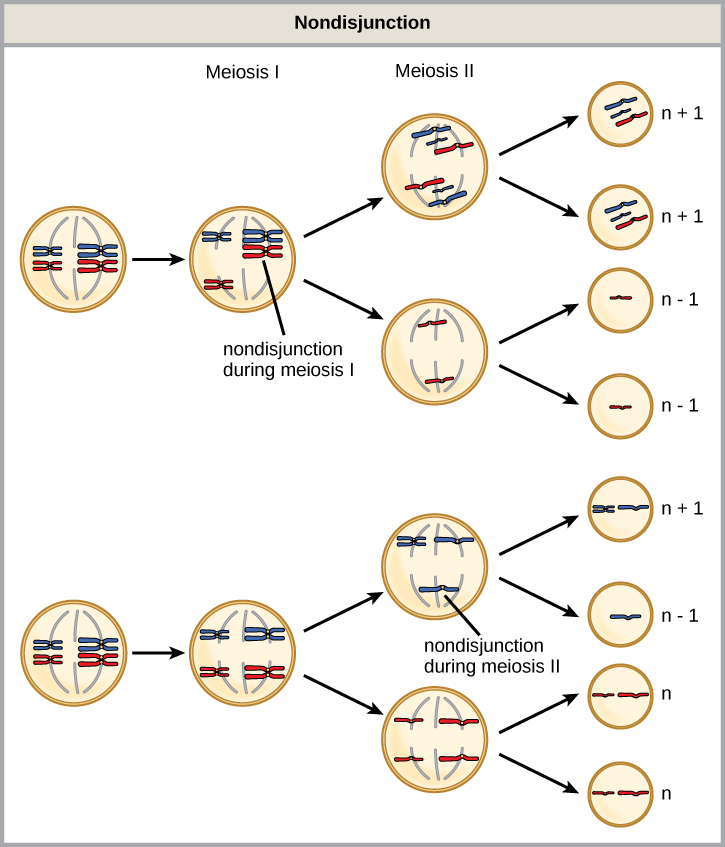
Which of the following statements about nondisjunction is true?
- Nondisjunction only results in gametes with n+1 or n–1 chromosomes.
- Nondisjunction occurring during meiosis II results in 50 percent normal gametes.
- Nondisjunction during meiosis I results in 50 percent normal gametes.
- Nondisjunction always results in four different kinds of gametes.
An individual with the appropriate number of chromosomes for their species is called euploid ; in humans, euploidy corresponds to 22 pairs of autosomes and one pair of sex chromosomes. An individual with an error in chromosome number is described as aneuploid , a term that includes monosomy (loss of one chromosome) or trisomy (gain of an extraneous chromosome). Monosomic human zygotes missing any one copy of an autosome invariably fail to develop to birth because they lack essential genes. This underscores the importance of “gene dosage” in humans. Most autosomal trisomies also fail to develop to birth; however, duplications of some of the smaller chromosomes (13, 15, 18, 21, or 22) can result in offspring that survive for several weeks to many years. Trisomic individuals suffer from a different type of genetic imbalance: an excess in gene dose. Individuals with an extra chromosome may synthesize an abundance of the gene products encoded by that chromosome. This extra dose (150 percent) of specific genes can lead to a number of functional challenges and often precludes development. The most common trisomy among viable births is that of chromosome 21, which corresponds to Down Syndrome. Individuals with this inherited disorder are characterized by short stature and stunted digits, facial distinctions that include a broad skull and large tongue, and significant developmental delays. The incidence of Down syndrome is correlated with maternal age; older women are more likely to become pregnant with fetuses carrying the trisomy 21 genotype (Figure \(\PageIndex{3}\)).
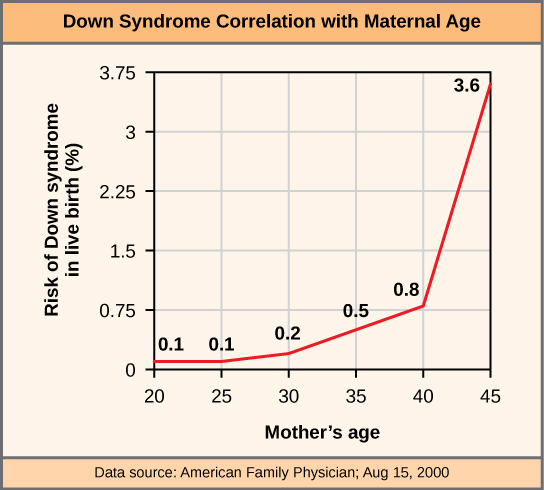
Link to Learning
Visualize the addition of a chromosome that leads to Down syndrome in this video simulation .
An individual with more than the correct number of chromosome sets (two for diploid species) is called polyploid . For instance, fertilization of an abnormal diploid egg with a normal haploid sperm would yield a triploid zygote. Polyploid animals are extremely rare, with only a few examples among the flatworms, crustaceans, amphibians, fish, and lizards. Polyploid animals are sterile because meiosis cannot proceed normally and instead produces mostly aneuploid daughter cells that cannot yield viable zygotes. Rarely, polyploid animals can reproduce asexually by haplodiploidy, in which an unfertilized egg divides mitotically to produce offspring. In contrast, polyploidy is very common in the plant kingdom, and polyploid plants tend to be larger and more robust than euploids of their species (Figure \(\PageIndex{4}\)).
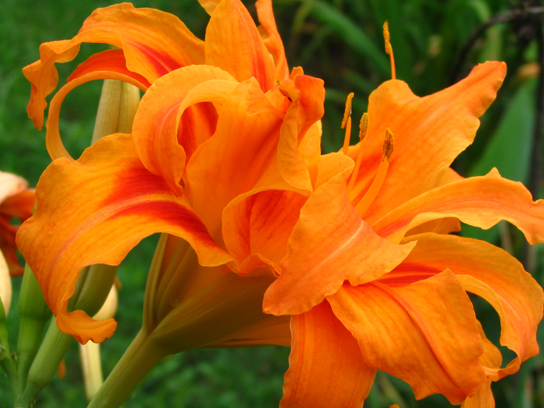
Sex Chromosome Nondisjunction in Humans
Humans display dramatic deleterious effects with autosomal trisomies and monosomies. Therefore, it may seem counterintuitive that human females and males can function normally, despite carrying different numbers of the X chromosome. Rather than a gain or loss of autosomes, variations in the number of sex chromosomes are associated with relatively mild effects. In part, this occurs because of a molecular process called X inactivation . Early in development, when female mammalian embryos consist of just a few thousand cells (relative to trillions in the newborn), one X chromosome in each cell inactivates by tightly condensing into a quiescent (dormant) structure called a Barr body. The chance that an X chromosome (maternally or paternally derived) is inactivated in each cell is random, but once the inactivation occurs, all cells derived from that one will have the same inactive X chromosome or Barr body. By this process, females compensate for their double genetic dose of X chromosome. In so-called “tortoiseshell” cats, embryonic X inactivation is observed as color variegation (Figure \(\PageIndex{5}\)). Females that are heterozygous for an X-linked coat color gene will express one of two different coat colors over different regions of their body, corresponding to whichever X chromosome is inactivated in the embryonic cell progenitor of that region.
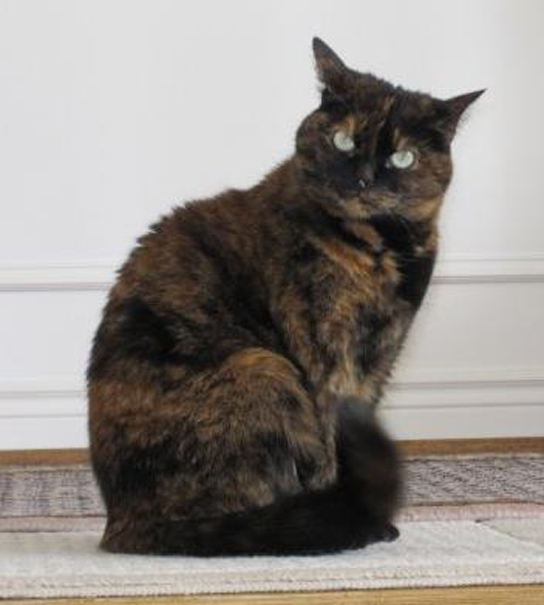
An individual carrying an abnormal number of X chromosomes will inactivate all but one X chromosome in each of her cells. However, even inactivated X chromosomes continue to express a few genes, and X chromosomes must reactivate for the proper maturation of female ovaries. As a result, X-chromosomal abnormalities are typically associated with mild mental and physical defects, as well as sterility. If the X chromosome is absent altogether, the individual will not develop in utero.
Several errors in sex chromosome number have been characterized. Individuals with three X chromosomes, called triplo-X, are phenotypically female but express developmental delays and reduced fertility. The XXY genotype, corresponding to one type of Klinefelter syndrome, corresponds to phenotypically male individuals with small testes, enlarged breasts, and reduced body hair. More complex types of Klinefelter syndrome exist in which the individual has as many as five X chromosomes. In all types, every X chromosome except one undergoes inactivation to compensate for the excess genetic dosage. This can be seen as several Barr bodies in each cell nucleus. Turner syndrome, characterized as an X0 genotype (i.e., only a single sex chromosome), corresponds to a phenotypically female individual with short stature, webbed skin in the neck region, hearing and cardiac impairments, and sterility.
Duplications and Deletions
In addition to the loss or gain of an entire chromosome, a chromosomal segment may be duplicated or lost. Duplications and deletions often produce offspring that survive but exhibit physical and mental abnormalities. Duplicated chromosomal segments may fuse to existing chromosomes or may be free in the nucleus. Cri-du-chat (from the French for “cry of the cat”) is a syndrome associated with nervous system abnormalities and identifiable physical features that result from a deletion of most of 5p (the small arm of chromosome 5) (Figure \(\PageIndex{6}\)). Infants with this genotype emit a characteristic high-pitched cry on which the disorder’s name is based.
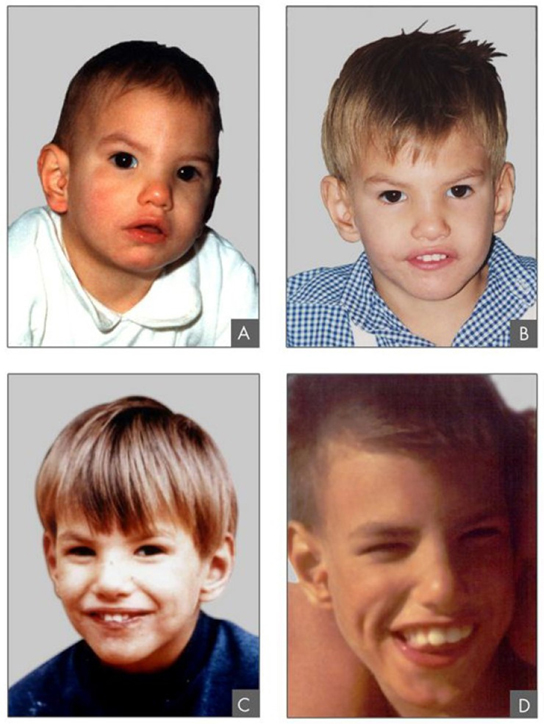
Chromosomal Structural Rearrangements
Cytologists have characterized numerous structural rearrangements in chromosomes, but chromosome inversions and translocations are the most common. Both are identified during meiosis by the adaptive pairing of rearranged chromosomes with their former homologs to maintain appropriate gene alignment. If the genes carried on two homologs are not oriented correctly, a recombination event could result in the loss of genes from one chromosome and the gain of genes on the other. This would produce aneuploid gametes.
Chromosome Inversions
A chromosome inversion is the detachment, 180° rotation, and reinsertion of part of a chromosome. Inversions may occur in nature as a result of mechanical shear, or from the action of transposable elements (special DNA sequences capable of facilitating the rearrangement of chromosome segments with the help of enzymes that cut and paste DNA sequences). Unless they disrupt a gene sequence, inversions only change the orientation of genes and are likely to have more mild effects than aneuploid errors. However, altered gene orientation can result in functional changes because regulators of gene expression could be moved out of position with respect to their targets, causing aberrant levels of gene products.
An inversion can be pericentric and include the centromere, or paracentric and occur outside of the centromere (). A pericentric inversion that is asymmetric about the centromere can change the relative lengths of the chromosome arms, making these inversions easily identifiable.
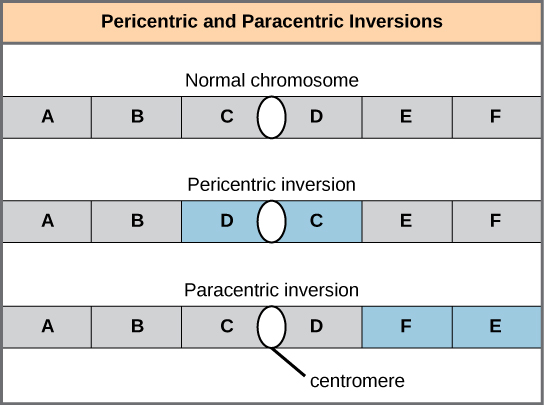
When one homologous chromosome undergoes an inversion but the other does not, the individual is described as an inversion heterozygote. To maintain point-for-point synapsis during meiosis, one homolog must form a loop, and the other homolog must mold around it. Although this topology can ensure that the genes are correctly aligned, it also forces the homologs to stretch and can be associated with regions of imprecise synapsis (Figure \(\PageIndex{8}\)).
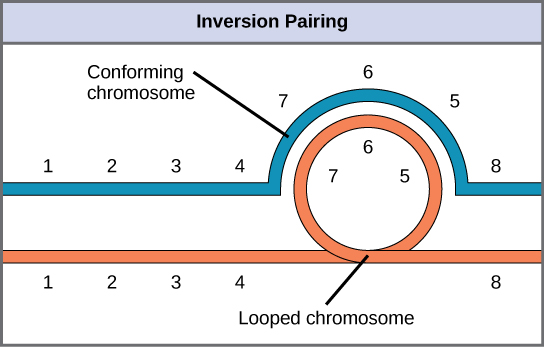
Evolution Connection: The Chromosome 18 Inversion
Not all structural rearrangements of chromosomes produce nonviable, impaired, or infertile individuals. In rare instances, such a change can result in the evolution of a new species. In fact, a pericentric inversion in chromosome 18 appears to have contributed to the evolution of humans. This inversion is not present in our closest genetic relatives, the chimpanzees. Humans and chimpanzees differ cytogenetically by pericentric inversions on several chromosomes and by the fusion of two separate chromosomes in chimpanzees that correspond to chromosome two in humans.
The pericentric chromosome 18 inversion is believed to have occurred in early humans following their divergence from a common ancestor with chimpanzees approximately five million years ago. Researchers characterizing this inversion have suggested that approximately 19,000 nucleotide bases were duplicated on 18p, and the duplicated region inverted and reinserted on chromosome 18 of an ancestral human.
A comparison of human and chimpanzee genes in the region of this inversion indicates that two genes— ROCK1 and USP14 —that are adjacent on chimpanzee chromosome 17 (which corresponds to human chromosome 18) are more distantly positioned on human chromosome 18. This suggests that one of the inversion breakpoints occurred between these two genes. Interestingly, humans and chimpanzees express USP14 at distinct levels in specific cell types, including cortical cells and fibroblasts. Perhaps the chromosome 18 inversion in an ancestral human repositioned specific genes and reset their expression levels in a useful way. Because both ROCK1 and USP14 encode cellular enzymes, a change in their expression could alter cellular function. It is not known how this inversion contributed to hominid evolution, but it appears to be a significant factor in the divergence of humans from other primates. 1
Translocations
A translocation occurs when a segment of a chromosome dissociates and reattaches to a different, nonhomologous chromosome. Translocations can be benign or have devastating effects depending on how the positions of genes are altered with respect to regulatory sequences. Notably, specific translocations have been associated with several cancers and with schizophrenia. Reciprocal translocations result from the exchange of chromosome segments between two nonhomologous chromosomes such that there is no gain or loss of genetic information (Figure \(\PageIndex{9}\)).
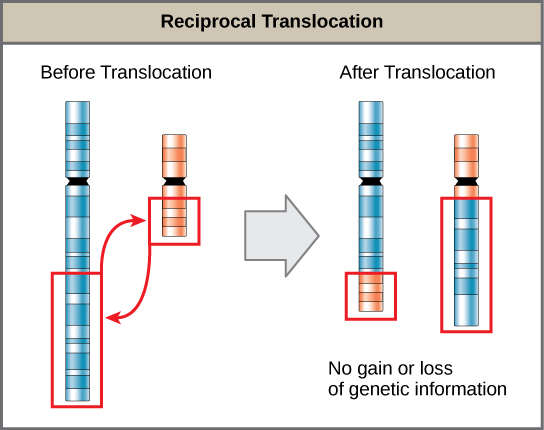
The number, size, shape, and banding pattern of chromosomes make them easily identifiable in a karyogram and allows for the assessment of many chromosomal abnormalities. Disorders in chromosome number, or aneuploidies, are typically lethal to the embryo, although a few trisomic genotypes are viable. Because of X inactivation, aberrations in sex chromosomes typically have milder phenotypic effects. Aneuploidies also include instances in which segments of a chromosome are duplicated or deleted. Chromosome structures may also be rearranged, for example by inversion or translocation. Both of these aberrations can result in problematic phenotypic effects. Because they force chromosomes to assume unnatural topologies during meiosis, inversions and translocations are often associated with reduced fertility because of the likelihood of nondisjunction.
Art Connections
Figure \(\PageIndex{2}\): Which of the following statements about nondisjunction is true?
- 1 Violaine Goidts et al., “Segmental duplication associated with the human-specific inversion of chromosome 18: a further example of the impact of segmental duplications on karyotype and genome evolution in primates,” Human Genetics . 115 (2004):116-122
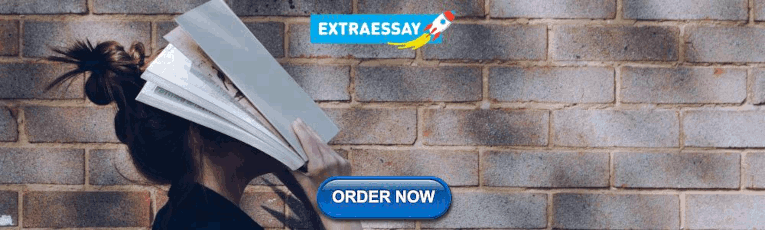
IMAGES
VIDEO
COMMENTS
Modes of Extrachromosomal Inheritance. 1. Uniparental inheritance. Uniparental inheritance refers to a mode of inheritance where genetic material or traits are inherited from a single parent, either the mother or the father. The genomes of extrachromosomal organelles are maternally inherited in most eukaryotes.
X-linked inheritance most often affects males due to a lack of a functional copy of the gene on the Y chromosome, though females may also be affected if the mutation causes a dominant-negative or gain-of-function effect, or inheritance of two affected X chromosomes occurs. ... or inheritance of two affected X chromosomes occurs. The most ...
cannot be accounted for on the basis of chromosomal genetics. The term cytoplasmic heredity is often used synonymously for extrachro mosomal inheritance, then, as a categorical alternative to chromosomal heredity. Since two or more different cytoplasmic organelles are already known to be implicated in genetic systems, the term covers a conglo
The extrachromosomal DNA is inherited from the maternal side because the female gamete contains more cytoplasm than the male gamete. 4. All the progenies obtained by this inheritance have the phenotype of only one parent, i.e., the mother. 5. The extranuclear genes present in the mitochondria and plastids cannot be mapped to the chromosomes in ...
The concept of genetic identity by descent is central to the application of evolutionary theory to cancer, suggesting a physical basis for identity through chromosomal inheritance during cell ...
The absence of Mendelian inheritance in cytoplasmic genomes likely contributes to the tremendous variation observed there, to the extent that once multi-chromosomal genomes evolve, their inheritance is highly fragmented and inconsistent (Wu and Sloan 2019). In parallel, heritable symbiont genomes vary greatly in genome complexity.
While the term genome includes all of the chromosomal hereditary determinants, the extrachromosomal hereditary ... The expressions" cytoplasmic" and" extra nuclear inheritance" as well as "extra chromosomal inheritance" have all been used in the literature. Vve prefer "extrachromosomal inheritance" in order to emphasize that the 439 K. Esser ...
Abstract. As early as 1909 Carl Correns, one of the rediscoverers of the Mendelian laws, recognized that the nucleus did not have a monopoly on heredity. He was able to show that the cytoplasm also carries hereditary determinants. In the years following, many cases of extrachromosomal inheritance were described in plants and animals.
Abstract. Most heritable information in eukaryotic cells is encoded in the nuclear genome, with inheritance patterns following classic Mendelian segregation. Genomes residing in the cytoplasm ...
That same year, Walter Sutton observed chromosome separation into daughter cells during meiosis (Figure 13.2). Together, these observations led to the Chromosomal Theory of Inheritance, which identified chromosomes as the genetic material responsible for Mendelian inheritance. Figure 13.2 (a) Walter Sutton and (b) Theodor Boveri developed the ...
Criteria for extrachromosomal inheritance: The extrachromosomal DNA follows a non-mendelian pattern of inheritance. Unlike the common nuclear DNA, genes present on organelle don't follow the Mendelian style of Inheritance. It is a circular genome, lacking centromere and that's why can't segregate like the genomic DNA.
Figure 13.1A. 1 13.1 A. 1: Sutton and Boveri: (a) Walter Sutton and (b) Theodor Boveri are credited with developing the Chromosomal Theory of Inheritance, which states that chromosomes carry the unit of heredity (genes). The Chromosomal Theory of Inheritance was consistent with Mendel's laws and was supported by the following observations:
Validation of the Chromosome Theory of Inheritance Thomas Hunt Morgan describes an X-linked gene for eye color in Drosophila. In 1910, a white-eyed male fly arose in a stock of red-eyed flies in Morgan's laboratory. Morgan called the gene responsible for eye color the white gene, because a mutation destroying its function gives white eyes.
In 1902 and 1903, Sutton and Boveri published independent papers proposing what we now call the chromosome theory of inheritance. This theory states that individual genes are found at specific locations on particular chromosomes, and that the behavior of chromosomes during meiosis can explain why genes are inherited according to Mendel's laws ...
M.M. Rhoades described another extrachromosomal inheritance in 1933. He proposed that male sterility in maize is regulated by maternal inheritance, and this became one of science's most important findings. Another feature that distinguishes extrachromosomal DNA is maternal inheritance. It is passed on from mother to kids.
The Chromosomal Theory of inheritance, proposed by Sutton and Boveri, states that chromosomes are the vehicles of genetic heredity. Neither Mendelian genetics nor gene linkage is perfectly accurate; instead, chromosome behavior involves segregation, independent assortment, and occasionally, linkage. Sturtevant devised a method to assess ...
You inherit one chromosome in each pair—a full complement of 23—from each parent. This occurs when the sperm and oocyte combine at the moment of your conception. Homologous chromosomes—those that make up a complementary pair—have genes for the same characteristics in the same location on the chromosome.
The chromosomal basis of inheritance provides an understanding of the pattern of transmission of genes from parent to offspring. SYI-3.C.3 Certain human genetic disorders can be attributed to the inheritance of a single affected or mutated allele or specific chromosomal changes, such as nondisjunction. 5.6 Chromosomal Inheritance Overview
Extra chromosomal inheritance defined as non-mendelian inheritance, usually involving DNA in replicating mitochondria and some other organelles of cell. Commonly defined as transmission through cytoplasm rather than nucleus. Plasma genes are located in DNA present in mitochondria and in chloroplast. Together both the DNAs are called organelle DNA.
This page titled 13.3: Chromosomal Basis of Inherited Disorders is shared under a CC BY 4.0 license and was authored, remixed, and/or curated by OpenStax via source content that was edited to the style and standards of the LibreTexts platform; a detailed edit history is available upon request. The number, size, shape, and banding pattern of ...
Together, these observations led to the development of the Chromosomal Theory of Inheritance, which identified chromosomes as the genetic material responsible for Mendelian inheritance. Figure \(\PageIndex{1}\): Sutton and Boveri: (a) Walter Sutton and (b) Theodor Boveri are credited with developing the Chromosomal Theory of Inheritance, which ...
The Chromosomal Theory of Inheritance. The chromosomal theory of inheritance was given by Boveri and Sutton in the early 1900s. It is the fundamental theory of genetics. According to this theory, genes are the units of heredity and are found in the chromosomes. Chromosomal Theory of Inheritance came into existence long after Mendelian genetics.
This page titled 12.2: Chromosomal Basis of Inherited Disorders is shared under a CC BY license and was authored, remixed, and/or curated by OpenStax. The number, size, shape, and banding pattern of chromosomes make them easily identifiable in a karyogram and allows for the assessment of many chromosomal abnormalities.