- Download Data Tables
- Download Study Aids
- Homework problem hints and answers
- Get Help from Dr. B in the LT Blog
- 120 day membership
Get it ALL for $5 US
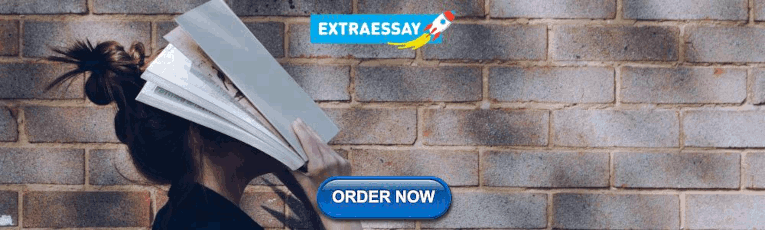
Thermodynamics Example Problems
- Some textbooks do not have enough example problems to help students learn how to solve problems.
- In other books, the examples do not teach the students the underlying method or approach to solving probelms.
- In many courses, the instructor posts copies of pages from the solution manual.
- Often the solution manual does little more than show the quickest way to obtain the answer and says nothing about WHY each step is taken or HOW the author knew which step to take next.
- Here you will find a hefty number of example problems worked out in great detail.
- I like to focus on HOW we know which step comes next and WHY certain equations or techniques apply.
- I hope you learn quickly and easily from these problems.

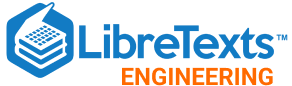
- school Campus Bookshelves
- menu_book Bookshelves
- perm_media Learning Objects
- login Login
- how_to_reg Request Instructor Account
- hub Instructor Commons
Margin Size
- Download Page (PDF)
- Download Full Book (PDF)
- Periodic Table
- Physics Constants
- Scientific Calculator
- Reference & Cite
- Tools expand_more
- Readability
selected template will load here
This action is not available.
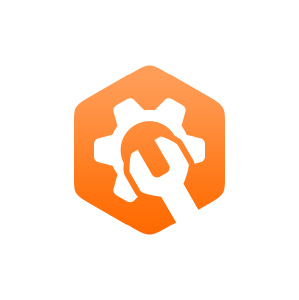
6.3: Refrigerator and heat pump
- Last updated
- Save as PDF
- Page ID 88853
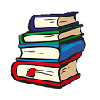
- Claire Yu Yan
- University of British Columbia, Okanagan via BC Campus
\( \newcommand{\vecs}[1]{\overset { \scriptstyle \rightharpoonup} {\mathbf{#1}} } \)
\( \newcommand{\vecd}[1]{\overset{-\!-\!\rightharpoonup}{\vphantom{a}\smash {#1}}} \)
\( \newcommand{\id}{\mathrm{id}}\) \( \newcommand{\Span}{\mathrm{span}}\)
( \newcommand{\kernel}{\mathrm{null}\,}\) \( \newcommand{\range}{\mathrm{range}\,}\)
\( \newcommand{\RealPart}{\mathrm{Re}}\) \( \newcommand{\ImaginaryPart}{\mathrm{Im}}\)
\( \newcommand{\Argument}{\mathrm{Arg}}\) \( \newcommand{\norm}[1]{\| #1 \|}\)
\( \newcommand{\inner}[2]{\langle #1, #2 \rangle}\)
\( \newcommand{\Span}{\mathrm{span}}\)
\( \newcommand{\id}{\mathrm{id}}\)
\( \newcommand{\kernel}{\mathrm{null}\,}\)
\( \newcommand{\range}{\mathrm{range}\,}\)
\( \newcommand{\RealPart}{\mathrm{Re}}\)
\( \newcommand{\ImaginaryPart}{\mathrm{Im}}\)
\( \newcommand{\Argument}{\mathrm{Arg}}\)
\( \newcommand{\norm}[1]{\| #1 \|}\)
\( \newcommand{\Span}{\mathrm{span}}\) \( \newcommand{\AA}{\unicode[.8,0]{x212B}}\)
\( \newcommand{\vectorA}[1]{\vec{#1}} % arrow\)
\( \newcommand{\vectorAt}[1]{\vec{\text{#1}}} % arrow\)
\( \newcommand{\vectorB}[1]{\overset { \scriptstyle \rightharpoonup} {\mathbf{#1}} } \)
\( \newcommand{\vectorC}[1]{\textbf{#1}} \)
\( \newcommand{\vectorD}[1]{\overrightarrow{#1}} \)
\( \newcommand{\vectorDt}[1]{\overrightarrow{\text{#1}}} \)
\( \newcommand{\vectE}[1]{\overset{-\!-\!\rightharpoonup}{\vphantom{a}\smash{\mathbf {#1}}}} \)
6.2.1 Refrigerator
A refrigerator is a cyclic device, which absorbs heat from a heat sink and reject heat to a heat source by consuming work. The working fluid is called refrigerant, which usually undergoes phase changes in the cycle.
A typical vapour-compression refrigeration system consists of mainly four pieces of equipment, as shown in Figure 6.2.1 :
- An evaporator, through which the low-pressure, low-temperature refrigerant absorbs heat from a heat sink (e.g., a freezer compartment or a space to be refrigerated) and changes from a two-phase mixture to a saturated or superheated vapour.
- A compressor, which is used to increase the pressure and temperature of the refrigerant vapour by consuming work.
- A condenser, through which heat is rejected to a heat source (e.g. kitchen or outdoor air). At the exit of the condenser, the refrigerant is typically a two-phase mixture or a liquid.
- An expansion valve, which is used to reduce the pressure and temperature of the refrigerant in order to achieve a liquid-vapour mixture of desirable quality at the exit of the expansion valve.
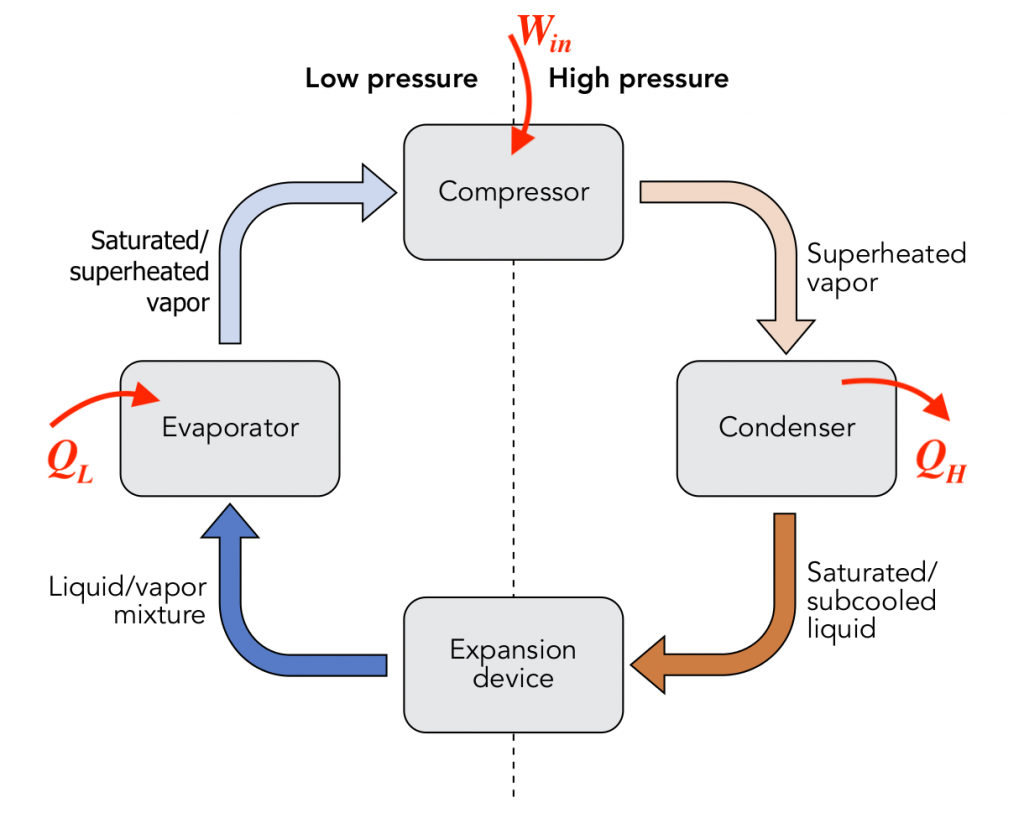
As heat cannot be transferred from a low-temperature body to a high-temperature body spontaneously in nature, refrigerators must consume work in order to operate between a heat sink and a heat source , even under ideal conditions.
Figure 6.2.2 is a schematic for analyzing the energy conservation in a refrigerator. The same schematic may be used to represent a heat pump by replacing the notation “Ref” with “HP” in the circular area.

Applying the first law of thermodynamics to the cycle, we can write
\[{\dot{Q}}_H-{\dot{Q}}_L = {\dot{W}}_{in}\]

6.2.2 Heat pump
A heat pump uses the same vapour compression refrigeration cycle, see Figure 6.2.1 , as a refrigerator. It absorbs heat from a heat sink (e.g., outdoor air in the winter) and delivers (more) heat to a heat source (e.g., indoor air) by consuming work. Applying the first law of thermodynamics to the heat pump cycle, we can derive
\[{\dot{W}}_{in}={\dot{Q}}_H-{\dot{Q}}_L\]

Consider a vapour-compression refrigeration cycle, Figure 6.2.1 . The working fluid is ammonia.

- At the compressor outlet, P 2 =1.4 MPa, T 2 =150 o C
- At the condenser outlet, P 3 =1.4 MPa, T 3 =35 o C
- At the evaporator inlet, P 4 =0.2 MPa

(1) The rates of heat transfer in the evaporator and condenser and the power consumption in the compressor depend on the changes of enthalpies in these devices. First, determine the specific enthalpy of each state from Tables B1 and B2.
- State 1, compressor inlet: P 1 = 0.2 MPa, T 1 = -10 o C. From Table B2 , h 1 = 1603.63 kJ/kg.
- State 2, compressor outlet: P 2 = 1.4 MPa, T 2 = 150 o C. From Table B2 , h 2 = 1940.26 kJ/kg
- State 3, condenser outlet: P 3 = 1.4 MPa, T 2 = 35 o C. From Table B1 , ammonia at this state is a compressed liquid; therefore, h 3 ≈ h f @35 o C = 509.23 kJ/kg
- State 4, evaporator inlet: P 4 = 0.2 MPa. Typically, the expansion valve is assumed well-insulated; therefore, the expansion process is adiabatic, and h 4 = h 3 = 509.24 kJ/kg.
The rate of the heat absorbed by the evaporator
\[\dot{Q}_{L} = \dot{m}(h_1 - h_4) = 0.1 \times (1603.63 - 509.23) = 109.44 \ kW\]
The power consumption by the compressor
\[\dot{W}_{in} = \dot{m}(h_2-h_1) = 0.1 \times(1940.26 - 1603.63) =33.66 \ kW\]
The rate of the heat rejected by the condenser
\[\dot{Q}_H = \dot{m}(h_2-h_3) = 0.1 \times(1940.26 - 509.23) = 143.10 \ kW\]

(2) COP of the refrigeration cycle
\[COP_R = \dfrac{\dot{Q}_{L}}{\dot{W}_{in}} = \dfrac{109.44}{33.66} = 3.25\]
(3) If the same cycle is used as a heat pump, its COP will be
\[COP_{HP}= \dfrac{\dot{Q}_{H}}{\dot{W}_{in}} = \dfrac{143.10}{33.66} = 4.25\]
\[COP_{HP}=COP_R+1\]
\[COP_{HP} = \dfrac{\dot{Q}_{H}}{\dot{W}_{in}} = \dfrac{\dot{Q}_{L} + \dot{W}_{in}}{\dot{W}_{in}} = COP_R + 1\]
Query \(\PageIndex{1}\)
Media Attributions
- Vapor compression refrigeration cycle © WGisol is licensed under a CC BY-SA (Attribution ShareAlike) license
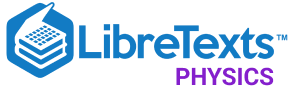
- school Campus Bookshelves
- menu_book Bookshelves
- perm_media Learning Objects
- login Login
- how_to_reg Request Instructor Account
- hub Instructor Commons
Margin Size
- Download Page (PDF)
- Download Full Book (PDF)
- Periodic Table
- Physics Constants
- Scientific Calculator
- Reference & Cite
- Tools expand_more
- Readability
selected template will load here
This action is not available.
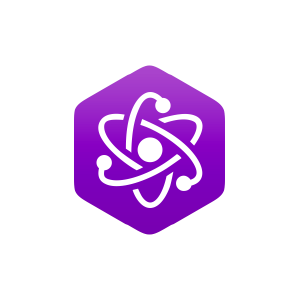
15.5: Applications of Thermodynamics- Heat Pumps and Refrigerators
- Last updated
- Save as PDF
- Page ID 1598
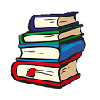
\( \newcommand{\vecs}[1]{\overset { \scriptstyle \rightharpoonup} {\mathbf{#1}} } \)
\( \newcommand{\vecd}[1]{\overset{-\!-\!\rightharpoonup}{\vphantom{a}\smash {#1}}} \)
\( \newcommand{\id}{\mathrm{id}}\) \( \newcommand{\Span}{\mathrm{span}}\)
( \newcommand{\kernel}{\mathrm{null}\,}\) \( \newcommand{\range}{\mathrm{range}\,}\)
\( \newcommand{\RealPart}{\mathrm{Re}}\) \( \newcommand{\ImaginaryPart}{\mathrm{Im}}\)
\( \newcommand{\Argument}{\mathrm{Arg}}\) \( \newcommand{\norm}[1]{\| #1 \|}\)
\( \newcommand{\inner}[2]{\langle #1, #2 \rangle}\)
\( \newcommand{\Span}{\mathrm{span}}\)
\( \newcommand{\id}{\mathrm{id}}\)
\( \newcommand{\kernel}{\mathrm{null}\,}\)
\( \newcommand{\range}{\mathrm{range}\,}\)
\( \newcommand{\RealPart}{\mathrm{Re}}\)
\( \newcommand{\ImaginaryPart}{\mathrm{Im}}\)
\( \newcommand{\Argument}{\mathrm{Arg}}\)
\( \newcommand{\norm}[1]{\| #1 \|}\)
\( \newcommand{\Span}{\mathrm{span}}\) \( \newcommand{\AA}{\unicode[.8,0]{x212B}}\)
\( \newcommand{\vectorA}[1]{\vec{#1}} % arrow\)
\( \newcommand{\vectorAt}[1]{\vec{\text{#1}}} % arrow\)
\( \newcommand{\vectorB}[1]{\overset { \scriptstyle \rightharpoonup} {\mathbf{#1}} } \)
\( \newcommand{\vectorC}[1]{\textbf{#1}} \)
\( \newcommand{\vectorD}[1]{\overrightarrow{#1}} \)
\( \newcommand{\vectorDt}[1]{\overrightarrow{\text{#1}}} \)
\( \newcommand{\vectE}[1]{\overset{-\!-\!\rightharpoonup}{\vphantom{a}\smash{\mathbf {#1}}}} \)
Learning Objectives
By the end of this section, you will be able to:
- Describe the use of heat engines in heat pumps and refrigerators.
- Demonstrate how a heat pump works to warm an interior space.
- Explain the differences between heat pumps and refrigerators.
- Calculate a heat pump’s coefficient of performance.

Heat pumps, air conditioners, and refrigerators utilize heat transfer from cold to hot. They are heat engines run backward. We say backward, rather than reverse, because except for Carnot engines, all heat engines, though they can be run backward, cannot truly be reversed. Heat transfer occurs from a cold reservoir \(Q_c\) and into a hot one. This requires work input \(W\), which is also converted to heat transfer. Thus the heat transfer to the hot reservoir is \(Q_h = Q_c + W\). (Note that \(Q_h\), \(Q_c\) and \(W\) are positive, with their directions indicated on schematics rather than by sign.) A heat pump’s mission is for heat transfer \(Q-h\) to occur into a warm environment, such as a home in the winter. The mission of air conditioners and refrigerators is for heat transfer \(Q_c\) to occur from a cool environment, such as chilling a room or keeping food at lower temperatures than the environment. (Actually, a heat pump can be used both to heat and cool a space. It is essentially an air conditioner and a heating unit all in one. In this section we will concentrate on its heating mode.)

The great advantage of using a heat pump to keep your home warm, rather than just burning fuel, is that a heat pump supplies \(Q_h = Q_c + W\). Heat transfer is from the outside air, even at a temperature below freezing, to the indoor space. You only pay for \(W\), and you get an additional heat transfer of \(Q_c\) from the outside at no cost; in many cases, at least twice as much energy is transferred to the heated space as is used to run the heat pump. When you burn fuel to keep warm, you pay for all of it. The disadvantage is that the work input (required by the second law of thermodynamics) is sometimes more expensive than simply burning fuel, especially if the work is done by electrical energy.
The basic components of a heat pump in its heating mode are shown in Figure \(\PageIndex{3}\). A working fluid such as a non-CFC refrigerant is used. In the outdoor coils (the evaporator), heat transfer \(Q_c\) occurs to the working fluid from the cold outdoor air, turning it into a gas.

The electrically driven compressor (work input \(W\)) raises the temperature and pressure of the gas and forces it into the condenser coils that are inside the heated space. Because the temperature of the gas is higher than the temperature inside the room, heat transfer to the room occurs and the gas condenses to a liquid. The liquid then flows back through a pressure-reducing valve to the outdoor evaporator coils, being cooled through expansion. (In a cooling cycle, the evaporator and condenser coils exchange roles and the flow direction of the fluid is reversed.)
The quality of a heat pump is judged by how much heat transfer \(Q_h\) occurs into the warm space compared with how much work input \(W\) is required. In the spirit of taking the ratio of what you get to what you spend, we define a heat pump’s coefficient of performance \((COP_{hp})\) to be \[COP_{hp} = \dfrac{Q_h}{W}.\] Since the efficiency of a heat engine is \(Eff = W/Q_h\), wee see that \(COP_{hp} = 1/Eff\), an important and interesting fact. First, since the efficiency of any heat engine is less than 1, it means that \(COP_{hp}\) is always greater than 1—that is, a heat pump always has more heat transfer \(Q_h\) than work put into it. Second, it means that heat pumps work best when temperature differences are small. The efficiency of a perfect, or Carnot, engine is \(Eff_c = 1 - (T_c?T_h)\) thus, the smaller the temperature difference, the smaller the efficiency and the greater the \(COP_{hp}\) (because \(COP_{hp} = 1/Eff\)). In other words, heat pumps do not work as well in very cold climates as they do in more moderate climates.
Friction and other irreversible processes reduce heat engine efficiency, but they do not benefit the operation of a heat pump—instead, they reduce the work input by converting part of it to heat transfer back into the cold reservoir before it gets into the heat pump.

Example \(\PageIndex{1}\): The Best \(COP_{hp}\) of a Heat Pump for Home Use
A heat pump used to warm a home must employ a cycle that produces a working fluid at temperatures greater than typical indoor temperature so that heat transfer to the inside can take place. Similarly, it must produce a working fluid at temperatures that are colder than the outdoor temperature so that heat transfer occurs from outside. Its hot and cold reservoir temperatures therefore cannot be too close, placing a limit on its \(COP{hp}\). (See Figure \(\PageIndex{5}\).) What is the best coefficient of performance possible for such a heat pump, if it has a hot reservoir temperature of \(45.0^oC\) and a cold reservoir temperature of \(-15.0^oC\)?
A Carnot engine reversed will give the best possible performance as a heat pump. As noted above, \(COP_{hp} = 1/Eff\), so that we need to first calculate the Carnot efficiency to solve this problem.
Carnot efficiency in terms of absolute temperature is given by : \[Eff_c = 1 - \dfrac{T_c}{T_h}.\]
The temperatures in kelvins are \(T_h = 318 \, K\) and \(T_c = 258 \, K\), so that \[Eff_c = 1 - \dfrac{258}{318} K = 0.1887.\]
Thus, from the discussion above, \[COP_{hp} = \dfrac{1}{0.1887} = 5.30,\] or \[COP_{hp} = \dfrac{Q_h}{W} = 5.30,\] so that \[Q_h = 5.30 \, W.\]
This result means that the heat transfer by the heat pump is 5.30 times as much as the work put into it. It would cost 5.30 times as much for the same heat transfer by an electric room heater as it does for that produced by this heat pump. This is not a violation of conservation of energy. Cold ambient air provides 4.3 J per 1 J of work from the electrical outlet.

Real heat pumps do not perform quite as well as the ideal one in the previous example; their values of \(COP_{hp}\) range from about 2 to 4. This range means that the heat transfer \(Q_h\) from the heat pumps is 2 to 4 times as great as the work \(W\) put into them. Their economical feasibility is still limited, however, since \(W\) is usually supplied by electrical energy that costs more per joule than heat transfer by burning fuels like natural gas. Furthermore, the initial cost of a heat pump is greater than that of many furnaces, so that a heat pump must last longer for its cost to be recovered. Heat pumps are most likely to be economically superior where winter temperatures are mild, electricity is relatively cheap, and other fuels are relatively expensive. Also, since they can cool as well as heat a space, they have advantages where cooling in summer months is also desired. Thus some of the best locations for heat pumps are in warm summer climates with cool winters. Figure \(\PageIndex{6}\) shows a heat pump, called a “ reverse cycle” or “ split-system cooler” in some countries.

Air Conditioners and Refrigerators
Air conditioners and refrigerators are designed to cool something down in a warm environment. As with heat pumps, work input is required for heat transfer from cold to hot, and this is expensive. The quality of air conditioners and refrigerators is judged by how much heat transfer \(Q_c\) occurs from a cold environment compared with how much work input \(W\) is required. What is considered the benefit in a heat pump is considered waste heat in a refrigerator. We thus define the coefficient of performance \(COP_{ref}\) of an air conditioner or refrigerator to be \[COP_{ref} = \dfrac{Q_c}{W}.\]
Noting again that \(Q_h = Q_c + W\), we can see that an air conditioner will have a lower coefficient of performance than a heat pump, because \(COP_{hp} = Q_h/W\) and \(Q_h\) is greater than \(Q_c\). In this module’s Problems and Exercises, you will show that \[COP_{ref} = COP_{hp} - 1\] for a heat engine used as either an air conditioner or a heat pump operating between the same two temperatures. Real air conditioners and refrigerators typically do remarkably well, having values of \(COP_{ref}\) ranging from 2 to 6. These numbers are better than the \(COP_{hp}\) values for the heat pumps mentioned above, because the temperature differences are smaller, but they are less than those for Carnot engines operating between the same two temperatures.
A type of \(COP\) rating system called the “energy efficiency rating” (\(EER\)) has been developed. This rating is an example where non-SI units are still used and relevant to consumers. To make it easier for the consumer, Australia, Canada, New Zealand, and the U.S. use an Energy Star Rating out of 5 stars—the more stars, the more energy efficient the appliance. \(EER\)s are expressed in mixed units of British thermal units (Btu) per hour of heating or cooling divided by the power input in watts. Room air conditioners are readily available with \(EER\)s ranging from 6 to 12. Although not the same as the \(COP\) just described, these \(EER\)s are good for comparison purposes—the greater the \(EER\), the cheaper an air conditioner is to operate (but the higher its purchase price is likely to be).
The \(EER\) of an air conditioner or refrigerator can be expressed as \[EER = \dfrac{Q_c/t_1}{W/t_2},\] where \(Q_c\) is the amount of heat transfer from a cold environment in British thermal units, \(t_1\) is time in hours, \(W\) is the work input in joules, and \(t_2\) is time in seconds.
PROBLEM SOLVING STRATEGIES FOR THERMODYNAMICS
- Examine the situation to determine whether heat, work, or internal energy are involved . Look for any system where the primary methods of transferring energy are heat and work. Heat engines, heat pumps, refrigerators, and air conditioners are examples of such systems.
- Identify the system of interest and draw a labeled diagram of the system showing energy flow.
- Identify exactly what needs to be determined in the problem (identify the unknowns) . A written list is useful. Maximum efficiency means a Carnot engine is involved. Efficiency is not the same as the coefficient of performance.
- Make a list of what is given or can be inferred from the problem as stated (identify the knowns) . Be sure to distinguish heat transfer into a system from heat transfer out of the system, as well as work input from work output. In many situations, it is useful to determine the type of process, such as isothermal or adiabatic.
- Solve the appropriate equation for the quantity to be determined (the unknown).
- Substitute the known quantities along with their units into the appropriate equation and obtain numerical solutions complete with units.
- Check the answer to see if it is reasonable: Does it make sense? For example, efficiency is always less than 1, whereas coefficients of performance are greater than 1.
- An artifact of the second law of thermodynamics is the ability to heat an interior space using a heat pump. Heat pumps compress cold ambient air and, in so doing, heat it to room temperature without violation of conservation principles.
- To calculate the heat pump’s coefficient of performance, use the equation \(COP_{hp} = \dfrac{Q_h}{W}\).
- A refrigerator is a heat pump; it takes warm ambient air and expands it to chill it.
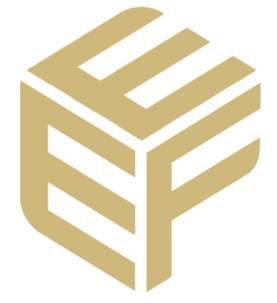
Refrigeration Cycle: Example Problem
Try to solve these problems before watching the solutions in the screencasts.
Example Problem 1
A refrigeration cycle using freon-12 is shown in the log pressure versus enthalpy plot. This cycle uses a throttle valve. The enthalpy and entropy values in the plot are from tables for freon-12. Calculate: 1. Heat transferred at low temperature and at high temperature. 2. Work input to refrigerator. 3. The coefficient of performance (COP) for the cycle. 4. The COP if the compressor is only 70% efficient.
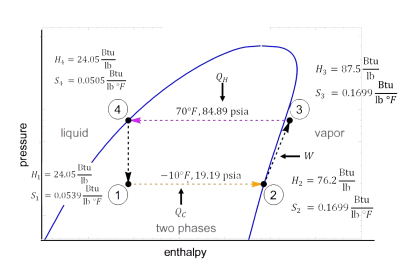
Example Problem 2
Consider a vapor-compression refrigeration cycle that uses R134a as the working fluid. The condenser, which yields saturated liquid, is operated at 45C; the evaporator, which yields saturated vapor, is operated at -10.C. The compressor is 80.% efficient. Using data from the table below, determine: 1. The amount of cooling per kg of R134a 2. The amount of heat rejected per kg of R134a 3. The coefficient of performance.
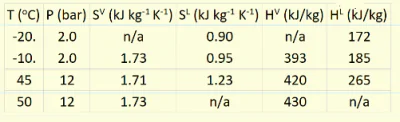
15.5 Applications of Thermodynamics: Heat Pumps and Refrigerators
Heat pumps, air conditioners, and refrigerators utilize heat transfer from cold to hot. They are heat engines run backward. We say backward, rather than reverse, because except for Carnot engines, all heat engines, though they can be run backward, cannot truly be reversed. Heat transfer occurs from a cold reservoir Q c Q c size 12{Q rSub { size 8{c} } } {} and into a hot one. This requires work input W W size 12{W} {} , which is also converted to heat transfer. Thus the heat transfer to the hot reservoir is Q h = Q c + W Q h = Q c + W size 12{Q rSub { size 8{h} } =Q rSub { size 8{c} } +W} {} . (Note that Q h Q h size 12{Q rSub { size 8{h} } } {} , Q c Q c size 12{Q rSub { size 8{c} } } {} , and W W size 12{W} {} are positive, with their directions indicated on schematics rather than by sign.) A heat pump’s mission is for heat transfer Q h Q h size 12{Q rSub { size 8{h} } } {} to occur into a warm environment, such as a home in the winter. The mission of air conditioners and refrigerators is for heat transfer Q c Q c size 12{Q rSub { size 8{c} } } {} to occur from a cool environment, such as chilling a room or keeping food at lower temperatures than the environment. (Actually, a heat pump can be used both to heat and cool a space. It is essentially an air conditioner and a heating unit all in one. In this section we will concentrate on its heating mode.)
The great advantage of using a heat pump to keep your home warm, rather than just burning fuel, is that a heat pump supplies Q h = Q c + W Q h = Q c + W size 12{Q rSub { size 8{h} } =Q rSub { size 8{c} } +W} {} . Heat transfer is from the outside air, even at a temperature below freezing, to the indoor space. You only pay for W W size 12{W} {} , and you get an additional heat transfer of Q c Q c size 12{Q rSub { size 8{c} } } {} from the outside at no cost; in many cases, at least twice as much energy is transferred to the heated space as is used to run the heat pump. When you burn fuel to keep warm, you pay for all of it. The disadvantage is that the work input (required by the second law of thermodynamics) is sometimes more expensive than simply burning fuel, especially if the work is done by electrical energy.
The basic components of a heat pump in its heating mode are shown in Figure 15.28 . A working fluid such as a non-CFC refrigerant is used. In the outdoor coils (the evaporator), heat transfer Q c Q c size 12{Q rSub { size 8{c} } } {} occurs to the working fluid from the cold outdoor air, turning it into a gas.
The electrically driven compressor (work input W W size 12{W} {} ) raises the temperature and pressure of the gas and forces it into the condenser coils that are inside the heated space. Because the temperature of the gas is higher than the temperature inside the room, heat transfer to the room occurs and the gas condenses to a liquid. The liquid then flows back through a pressure-reducing valve to the outdoor evaporator coils, being cooled through expansion. (In a cooling cycle, the evaporator and condenser coils exchange roles and the flow direction of the fluid is reversed.)
The quality of a heat pump is judged by how much heat transfer Q h Q h size 12{Q rSub { size 8{h} } } {} occurs into the warm space compared with how much work input W W size 12{W} {} is required. In the spirit of taking the ratio of what you get to what you spend, we define a heat pump’s coefficient of performance ( COP hp COP hp size 12{ ital "COP" rSub { size 8{"hp"} } } {} ) to be
Since the efficiency of a heat engine is Eff = W / Q h Eff = W / Q h size 12{ ital "Eff"=W/Q rSub { size 8{h} } } {} , we see that COP hp = 1 / Eff COP hp = 1 / Eff size 12{ ital "COP" rSub { size 8{"hp"} } =1/ ital "Eff"} {} , an important and interesting fact. First, since the efficiency of any heat engine is less than 1, it means that COP hp COP hp size 12{ ital "COP" rSub { size 8{"hp"} } } {} is always greater than 1—that is, a heat pump always has more heat transfer Q h Q h size 12{Q rSub { size 8{h} } } {} than work put into it. Second, it means that heat pumps work best when temperature differences are small. The efficiency of a perfect, or Carnot, engine is Eff C = 1 − T c / T h Eff C = 1 − T c / T h size 12{ ital "Eff" rSub { size 8{C} } =1 - left (T rSub { size 8{c} } /T rSub { size 8{h} } right )} {} ; thus, the smaller the temperature difference, the smaller the efficiency and the greater the COP hp COP hp size 12{ ital "COP" rSub { size 8{"hp"} } } {} (because COP hp = 1 / Eff COP hp = 1 / Eff size 12{ ital "COP" rSub { size 8{"hp"} } =1/ ital "Eff"} {} ). In other words, heat pumps do not work as well in very cold climates as they do in more moderate climates.
Friction and other irreversible processes reduce heat engine efficiency, but they do not benefit the operation of a heat pump—instead, they reduce the work input by converting part of it to heat transfer back into the cold reservoir before it gets into the heat pump.
Example 15.5
The best cop hp of a heat pump for home use.
A heat pump used to warm a home must employ a cycle that produces a working fluid at temperatures greater than typical indoor temperature so that heat transfer to the inside can take place. Similarly, it must produce a working fluid at temperatures that are colder than the outdoor temperature so that heat transfer occurs from outside. Its hot and cold reservoir temperatures therefore cannot be too close, placing a limit on its COP hp COP hp size 12{ ital "COP" rSub { size 8{"hp"} } } {} . (See Figure 15.30 .) What is the best coefficient of performance possible for such a heat pump, if it has a hot reservoir temperature of 45 . 0 º C 45 . 0 º C size 12{"45" "." 0°C} {} and a cold reservoir temperature of − 15 . 0 º C − 15 . 0 º C size 12{-"15" "." 0°C} {} ?
A Carnot engine reversed will give the best possible performance as a heat pump. As noted above, COP hp = 1 / Eff COP hp = 1 / Eff size 12{ ital "COP" rSub { size 8{"hp"} } =1/ ital "Eff"} {} , so that we need to first calculate the Carnot efficiency to solve this problem.
Carnot efficiency in terms of absolute temperature is given by :
The temperatures in kelvins are T h = 318 K T h = 318 K size 12{T rSub { size 8{h} } ="318"" K"} {} and T c = 258 K T c = 258 K size 12{T rSub { size 8{c} } ="258"" K"} {} , so that
Thus, from the discussion above,
This result means that the heat transfer by the heat pump is 5.30 times as much as the work put into it. It would cost 5.30 times as much for the same heat transfer by an electric room heater as it does for that produced by this heat pump. This is not a violation of conservation of energy. Cold ambient air provides 4.3 J per 1 J of work from the electrical outlet.
Real heat pumps do not perform quite as well as the ideal one in the previous example; their values of COP hp COP hp size 12{ ital "COP" rSub { size 8{"hp"} } } {} range from about 2 to 4. This range means that the heat transfer Q h Q h size 12{Q rSub { size 8{h} } } {} from the heat pumps is 2 to 4 times as great as the work W W size 12{W} {} put into them. Their economical feasibility is still limited, however, since W W size 12{W} {} is usually supplied by electrical energy that costs more per joule than heat transfer by burning fuels like natural gas. Furthermore, the initial cost of a heat pump is greater than that of many furnaces, so that a heat pump must last longer for its cost to be recovered. Heat pumps are most likely to be economically superior where winter temperatures are mild, electricity is relatively cheap, and other fuels are relatively expensive. Also, since they can cool as well as heat a space, they have advantages where cooling in summer months is also desired. Thus some of the best locations for heat pumps are in warm summer climates with cool winters. Figure 15.31 shows a heat pump, called a “ reverse cycle” or “ split-system cooler” in some countries.
Air Conditioners and Refrigerators
Air conditioners and refrigerators are designed to cool something down in a warm environment. As with heat pumps, work input is required for heat transfer from cold to hot, and this is expensive. The quality of air conditioners and refrigerators is judged by how much heat transfer Q c Q c size 12{Q rSub { size 8{c} } } {} occurs from a cold environment compared with how much work input W W size 12{W} {} is required. What is considered the benefit in a heat pump is considered waste heat in a refrigerator. We thus define the coefficient of performance (COP ref ) (COP ref ) size 12{ ital "COP" rSub { size 8{"ref"} } } {} of an air conditioner or refrigerator to be
Noting again that Q h = Q c + W Q h = Q c + W size 12{Q rSub { size 8{h} } =Q rSub { size 8{c} } +W} {} , we can see that an air conditioner will have a lower coefficient of performance than a heat pump, because COP hp = Q h / W COP hp = Q h / W size 12{ ital "COP" rSub { size 8{"hp"} } =Q rSub { size 8{h} } /W} {} and Q h Q h size 12{Q rSub { size 8{h} } } {} is greater than Q c Q c size 12{Q rSub { size 8{c} } } {} . In this module’s Problems and Exercises, you will show that
for a heat engine used as either an air conditioner or a heat pump operating between the same two temperatures. Real air conditioners and refrigerators typically do remarkably well, having values of COP ref COP ref size 12{ ital "COP" rSub { size 8{"ref"} } } {} ranging from 2 to 6. These numbers are better than the COP hp COP hp size 12{ ital "COP" rSub { size 8{"hp"} } } {} values for the heat pumps mentioned above, because the temperature differences are smaller, but they are less than those for Carnot engines operating between the same two temperatures.
A type of COP COP size 12{ ital "COP"} {} rating system called the “energy efficiency rating” ( EER EER size 12{ ital "EER"} {} ) has been developed. This rating is an example where non-SI units are still used and relevant to consumers. To make it easier for the consumer, Australia, Canada, New Zealand, and the U.S. use an Energy Star Rating out of 5 stars—the more stars, the more energy efficient the appliance. EER s EER s size 12{ ital "EER"} {} are expressed in mixed units of British thermal units (Btu) per hour of heating or cooling divided by the power input in watts. Room air conditioners are readily available with EER s EER s size 12{ ital "EER"} {} ranging from 6 to 12. Although not the same as the COP s COP s size 12{ ital "COP"} {} just described, these EER s EER s size 12{ ital "EER"} {} are good for comparison purposes—the greater the EER EER size 12{ ital "EER"} {} , the cheaper an air conditioner is to operate (but the higher its purchase price is likely to be).
The EER EER { ital "EER"s} {} of an air conditioner or refrigerator can be expressed as
where Q c Q c {Q rSub { {c} } } {} is the amount of heat transfer from a cold environment in British thermal units, t 1 t 1 {Q rSub { {c} } } {} is time in hours, W W {W} {} is the work input in joules, and t 2 t 2 is time in seconds.
Problem-Solving Strategies for Thermodynamics
- Examine the situation to determine whether heat, work, or internal energy are involved . Look for any system where the primary methods of transferring energy are heat and work. Heat engines, heat pumps, refrigerators, and air conditioners are examples of such systems.
- Identify the system of interest and draw a labeled diagram of the system showing energy flow.
- Identify exactly what needs to be determined in the problem (identify the unknowns) . A written list is useful. Maximum efficiency means a Carnot engine is involved. Efficiency is not the same as the coefficient of performance.
- Make a list of what is given or can be inferred from the problem as stated (identify the knowns) . Be sure to distinguish heat transfer into a system from heat transfer out of the system, as well as work input from work output. In many situations, it is useful to determine the type of process, such as isothermal or adiabatic.
- Solve the appropriate equation for the quantity to be determined (the unknown).
- Substitute the known quantities along with their units into the appropriate equation and obtain numerical solutions complete with units.
- Check the answer to see if it is reasonable: Does it make sense? For example, efficiency is always less than 1, whereas coefficients of performance are greater than 1.
As an Amazon Associate we earn from qualifying purchases.
This book may not be used in the training of large language models or otherwise be ingested into large language models or generative AI offerings without OpenStax's permission.
Want to cite, share, or modify this book? This book uses the Creative Commons Attribution License and you must attribute OpenStax.
Access for free at https://openstax.org/books/college-physics/pages/1-introduction-to-science-and-the-realm-of-physics-physical-quantities-and-units
- Authors: Paul Peter Urone, Roger Hinrichs
- Publisher/website: OpenStax
- Book title: College Physics
- Publication date: Jun 21, 2012
- Location: Houston, Texas
- Book URL: https://openstax.org/books/college-physics/pages/1-introduction-to-science-and-the-realm-of-physics-physical-quantities-and-units
- Section URL: https://openstax.org/books/college-physics/pages/15-5-applications-of-thermodynamics-heat-pumps-and-refrigerators
© Mar 3, 2022 OpenStax. Textbook content produced by OpenStax is licensed under a Creative Commons Attribution License . The OpenStax name, OpenStax logo, OpenStax book covers, OpenStax CNX name, and OpenStax CNX logo are not subject to the Creative Commons license and may not be reproduced without the prior and express written consent of Rice University.
CHAPTER. 2 THERMODYNAMICS AND REFRIGERATION CYCLES
Thermodynamics is the study of energy, its transformations, and its relation to states of matter. This chapter covers the application of thermodynamics to refrigeration cycles. The first part reviews the first and second laws of thermodynamics and presents methods for calculating thermodynamic properties. The second and third parts address compression and absorption refrigeration cycles, two common methods of thermal energy transfer. The remaining parts present other methods of refrigeration cycles.
1. THERMODYNAMICS
A thermodynamic system is a region in space or a quantity of matter bounded by a closed surface. The surroundings include everything external to the system, and the system is separated from the surroundings by the system boundaries. These boundaries can be movable or fixed, real or imaginary.
Entropy and energy are important in any thermodynamic system. Entropy measures the molecular disorder of a system. The more mixed a system, the greater its entropy; an orderly or unmixed configuration is one of low entropy. Energy has the capacity for producing an effect and can be categorized into either stored or transient forms.
1.1 STORED ENERGY
Thermal (internal) energy is caused by the motion of molecules and/or intermolecular forces.
Potential energy (PE) is caused by attractive forces existing between molecules, or the elevation of the system.
Kinetic energy (KE) is the energy caused by the velocity of molecules and is expressed as
where V is the velocity of a fluid stream crossing the system boundary.
Chemical energy is caused by the arrangement of atoms composing the molecules.
Nuclear (atomic) energy derives from the cohesive forces holding protons and neutrons together as the atom’s nucleus.
1.2 ENERGY IN TRANSITION
Heat Q is the mechanism that transfers energy across the boundaries of systems with differing temperatures, always toward the lower temperature. Heat is positive when energy is added to the system (see Figure 1 ).
Work is the mechanism that transfers energy across the boundaries of systems with differing pressures (or force of any kind), always toward the lower pressure. If the total effect produced in the system can be reduced to the raising of a weight, then nothing but work has crossed the boundary. Work is positive when energy is removed from the system (see Figure 1 ).
Mechanical or shaft work W is the energy delivered or absorbed by a mechanism, such as a turbine, air compressor, or internal combustion engine.
Figure 1. Energy Flows in General Thermodynamic System
Flow work is energy carried into or transmitted across the system boundary because a pumping process occurs somewhere outside the system, causing fluid to enter the system. It can be more easily understood as the work done by the fluid just outside the system on the adjacent fluid entering the system to force or push it into the system. Flow work also occurs as fluid leaves the system.
where p is pressure and v is specific volume, or the volume displaced per unit mass evaluated at the inlet or exit.
A property of a system is any observable characteristic of the system. The state of a system is defined by specifying the minimum set of independent properties. The most common thermodynamic properties are temperature T , pressure p , and specific volume v or density ρ. Additional thermodynamic properties include entropy, stored forms of energy, and enthalpy.
Frequently, thermodynamic properties combine to form other properties. Enthalpy h is an important property that includes internal energy and flow work and is defined as
where u is the internal energy per unit mass.
Each property in a given state has only one definite value, and any property always has the same value for a given state, regardless of how the substance arrived at that state.
A process is a change in state that can be defined as any change in the properties of a system. A process is described by specifying the initial and final equilibrium states, the path (if identifiable), and the interactions that take place across system boundaries during the process.
A cycle is a process or a series of processes wherein the initial and final states of the system are identical. Therefore, at the conclusion of a cycle, all the properties have the same value they had at the beginning. Refrigerant circulating in a closed system undergoes a cycle.
A pure substance has a homogeneous and invariable chemical composition. It can exist in more than one phase, but the chemical composition is the same in all phases.
If a substance is liquid at the saturation temperature and pressure, it is called a saturated liquid . If the temperature of the liquid is lower than the saturation temperature for the existing pressure, it is called either a subcooled liquid (the temperature is lower than the saturation temperature for the given pressure) or a compressed liquid (the pressure is greater than the saturation pressure for the given temperature). Pressure and temperature of a subcooled liquid are independent properties because the temperature can increase while pressure remains constant.
When a substance exists as part liquid and part vapor at the saturation temperature, its quality is defined as the ratio of the mass of vapor to the total mass. Quality has meaning only when the substance is saturated (i.e., at saturation pressure and temperature). Pressure and temperature of saturated pure substances and azeotropic mixtures are not independent properties. For zeotropic mixtures, pressure and temperature are independent properties even at saturation condition.
According to the thermodynamic state postulate, the thermodynamic state of a simple, compressible system containing a pure substance can be fixed by knowing the values of two independent, intensive properties. For pure substances and azeotropic mixtures, the saturation temperature has a single value for a given pressure, or, the saturation pressure has a single value for a given temperature. This means that pressure and temperature are not independent intensive properties at saturation condition. Therefore, at saturation condition, the value of another intensive property-in addition to temperature or pressure-such as the quality or specific volume needs to be known to fix the thermodynamic state. For zeotropic mixtures, at a given pressure, the bubble point temperature and dew point temperature are different. Similarly, at a given temperature, the bubble point pressure and dew point pressure are different. Therefore, at saturation condition, temperature and pressure can serve as the two independent intensive properties, whose values once determined, are sufficient to fix the thermodynamic state of the system. For a pure substance and azeotropic mixture, the bubble point and dew point temperatures for a given pressure are equal, so are the bubble point and dew point pressures for a given temperature.
If a substance exists as a vapor at saturation temperature and pressure, it is called a saturated vapor . (Sometimes the term dry saturated vapor is used to emphasize that the quality is 100%.) When the vapor is at a temperature greater than the saturation temperature, it is a superheated vapor . Pressure and temperature of a superheated vapor are independent properties, because the temperature can increase while pressure remains constant. Gases such as air at room temperature and pressure are highly superheated vapors.
1.3 FIRST LAW OF THERMODYNAMICS
The first law of thermodynamics is often called the law of conservation of energy . The following form of the first-law equation is valid only in the absence of a nuclear or chemical reaction.
Based on the first law or the law of conservation of energy, for any system, open or closed, there is an energy balance as
Figure 1 illustrates energy flows into and out of a thermodynamic system. For the general case of multiple mass flows with uniform properties in and out of the system, the energy balance can be written
where subscripts i and f refer to the initial and final states, respectively.
Nearly all important engineering processes are commonly modeled as steady-flow processes. Steady flow signifies that all quantities associated with the system do not vary with time. Consequently,
where h ≡ u + pv as described in Equation (4) .
A second common application is the closed stationary system for which the first law equation reduces to
1.4 SECOND LAW OF THERMODYNAMICS
The second law of thermodynamics differentiates and quantifies processes that only proceed in a certain direction (irreversible) from those that are reversible. The second law may be described in several ways. One method uses the concept of entropy flow in an open system and the irreversibility associated with the process. The concept of irreversibility provides added insight into the operation of cycles. For example, the larger the irreversibility in a refrigeration cycle operating with a given refrigeration load between two fixed temperature levels, the larger the amount of work required to operate the cycle. Irreversibilities include pressure drops in lines and heat exchangers, heat transfer between fluids of different temperature, and mechanical friction. Reducing total irreversibility in a cycle improves cycle performance. In the limit of no irreversibilities, a cycle attains its maximum ideal efficiency.
In an open system, the second law of thermodynamics can be described in terms of entropy as
Equation (8) accounts for all entropy changes in the system. Rearranged, this equation becomes
In integrated form, if inlet and outlet properties, mass flow, and interactions with the surroundings do not vary with time, the general equation for the second law is
In many applications, the process can be considered to operate steadily with no change in time. The change in entropy of the system is therefore zero. The irreversibility rate , which is the rate of entropy production caused by irreversibilities in the process, can be determined by rearranging Equation (10) :
Equation (6) can be used to replace the heat transfer quantity. Note that the absolute temperature of the surroundings with which the system is exchanging heat is used in the last term. If the temperature of the surroundings is equal to the system temperature, heat is transferred reversibly and the last term in Equation (11) equals zero.
Equation (11) is commonly applied to a system with one mass flow in, the same mass flow out, no work, and negligible kinetic or potential energy flows. Combining Equations (6) and (11) yields
For closed systems, the second law equation reduces to
In a cycle, the reduction of work produced by a power cycle (or the increase in work required by a refrigeration cycle) equals the absolute ambient temperature multiplied by the sum of irreversibilities in all processes in the cycle. Thus, the difference in reversible and actual work for any refrigeration cycle, theoretical or real, operating under the same conditions, becomes
In second law equations, the value of temperature T must always be substituted in the absolute scale (i.e., degrees Rankine) .
Another second-law method to describe performance of engineering devices is the concept of exergy (also called the availability , potential energy , or work potential ), which is the maximum useful work that could be obtained from the system at a given state in a specified environment. There is always a difference between exergy and the actual work delivered by a device; this difference represents the room for improvement. Note that exergy is a property of the system/environment combination and not of the system alone. The exergy of a system in equilibrium with its environment is zero. The state of the environment is referred to as the dead state , because the system cannot do any work.
Exergy transfer is in three forms (heat, work, and mass flow), and is given by
where ψ = ( h – h 0 ) – T 0 ( s – s 0 ) + ( V 2 /2) + gz is flow exergy. Quantities with 0 as subscript represent the reference state. T is the temperature of the heat source from which heat Q is transferred to the system. V is the velocity of the system, used to calculate the kinetic energy of the system.
Exergy balance for any system undergoing any process can be expressed as
Taking the positive direction of heat transfer as to the system and the positive direction of work transfer as from the system, the general exergy balance relations can be expressed explicitly as
Here, V i and V f are initial and final volumes of the system.
1.5 THERMODYNAMIC ANALYSIS OF REFRIGERATION CYCLES
Refrigeration cycles transfer thermal energy from a region of low temperature T R to one of higher temperature. Usually the higher-temperature heat sink is the ambient air or cooling water, at temperature T 0 , the temperature of the surroundings.
The first and second laws of thermodynamics can be applied to individual components to determine mass and energy balances and the irreversibility of the components. This procedure is shown in later sections in this chapter.
Performance of a refrigeration cycle is usually described by a coefficient of performance (COP) , defined as the benefit of the cycle (amount of heat removed) divided by the required energy input to operate the cycle:
For a mechanical vapor compression system, the net energy supplied is usually in the form of work, mechanical or electrical, and may include work to the compressor and fans or pumps. Thus, for a vapor compression air-conditioning or refrigeration system intended to perform cooling operation,
whereas, for a vapor compression heat pump system intended to perform heating operation,
In an absorption refrigeration cycle, the net energy supplied is usually in the form of heat into the generator and work into the pumps and fans, or
Similarly, in an absorption/adsorption heat pump cycle intended to perform heating operation,
In many cases, work supplied to an absorption system is very small compared to the amount of heat supplied to the generator, so the work term is often neglected.
Applying the second law to an entire refrigeration cycle shows that a completely reversible cycle operating under the same conditions has the maximum possible COP. Departure of the actual cycle from an ideal reversible cycle is given by the refrigerating efficiency :
The Carnot cycle usually serves as the ideal reversible refrigeration cycle. For multistage cycles, each stage is described by a reversible cycle.
1.6 EQUATIONS OF STATE
The equation of state of a pure substance is a mathematical relation between pressure, specific volume, and temperature. When the system is in thermodynamic equilibrium,
The principles of statistical mechanics are used to (1) explore the fundamental properties of matter, (2) predict an equation of state based on the statistical nature of a particular system, or (3) propose a functional form for an equation of state with unknown parameters that are determined by measuring thermodynamic properties of a substance. A fundamental equation with this basis is the virial equation , which is expressed as an expansion in pressure p or in reciprocal values of volume per unit mass v as
where coefficients B′ , C′ , D′ , etc., and B , C , D , etc., are the virial coefficients. B′ and B are the second virial coefficients; C′ and C are the third virial coefficients, etc. The virial coefficients are functions of temperature only, and values of the respective coefficients in Equations (22) and (23) are related. For example, B′ = B / RT and C′ = ( C – B 2 )/( RT ) 2 .
The quantity pv / RT is also called the compressibility factor Z , or
An advantage of the virial form is that statistical mechanics can be used to predict the lower-order coefficients and provide physical significance to the virial coefficients. For example, in Equation (25) , the term B / v is a function of interactions between two molecules, C / v 2 between three molecules, etc. Because lower-order interactions are common, contributions of the higher-order terms are successively less. Thermodynamicists use the partition or distribution function to determine virial coefficients; however, experimental values of the second and third coefficients are preferred. For dense fluids, many higher-order terms are necessary that can neither be satisfactorily predicted from theory nor determined from experimental measurements. In general, a truncated virial expansion of four terms is valid for densities of less than one-half the value at the critical point. For higher densities, additional terms can be used and determined empirically.
Computers allow the use of very complex equations of state in calculating p-v-T values, even to high densities. The Benedict-Webb-Rubin (B-W-R) equation of state (Benedict et al. 1940) and Martin-Hou equation (1955) have had considerable use, but should generally be limited to densities less than the critical value. Strobridge (1962) suggested a modified Benedict-Webb-Rubin relation that gives excellent results at higher densities and can be used for a p-v-T surface that extends into the liquid phase.
The B-W-R equation has been used extensively for hydrocarbons (Cooper and Goldfrank 1967):
where the constant coefficients are A o , B o , C o , a , b , c , α, and γ.
The Martin-Hou equation, developed for fluorinated hydrocarbon properties, has been used to calculate the thermodynamic property tables in Chapter 30 and in ASHRAE Thermodynamic Properties of Refrigerants (Stewart et al. 1986). The Martin-Hou equation is
where the constant coefficients are A i , B i , C i , k , b , and a .
Strobridge (1962) suggested an equation of state that was developed for nitrogen properties and used for most cryogenic fluids. This equation combines the B-W-R equation of state with an equation for high-density nitrogen suggested by Benedict (1937). These equations have been used successfully for liquid and vapor phases, extending in the liquid phase to the triple-point temperature and the freezing line, and in the vapor phase from 18 to 1800°R , with pressures to 150,000 psi . The Strobridge equation is accurate within the uncertainty of the measured p-v-T data:
The 15 coefficients of this equation’s linear terms are determined by a least-square fit to experimental data. Hust and McCarty (1967) and Hust and Stewart (1966) give further information on methods and techniques for determining equations of state.
In the absence of experimental data, van der Waals’ principle of corresponding states can predict fluid properties. This principle relates properties of similar substances by suitable reducing factors (i.e., the p-v-T surfaces of similar fluids in a given region are assumed to be of similar shape). The critical point can be used to define reducing parameters to scale the surface of one fluid to the dimensions of another. Modifications of this principle, as suggested by Kamerlingh Onnes, a Dutch cryogenic researcher, have been used to improve correspondence at low pressures. The principle of corresponding states provides useful approximations, and numerous modifications have been reported. More complex treatments for predicting properties, which recognize similarity of fluid properties, are by generalized equations of state. These equations ordinarily allow adjustment of the p-v-T surface by introducing parameters. One example (Hirschfelder et al. 1958) allows for departures from the principle of corresponding states by adding two correlating parameters.
1.7 CALCULATING THERMODYNAMIC PROPERTIES
Although equations of state provide p-v-T relations , thermodynamic analysis usually requires values for internal energy, enthalpy, and entropy. These properties have been tabulated for many substances, including refrigerants (see Chapters 1 , 30 , and 33 ), and can be extracted from such tables by interpolating manually or with a suitable computer program. This approach is appropriate for hand calculations and for relatively simple computer models; however, for many computer simulations, the overhead in memory or input and output required to use tabulated data can make this approach unacceptable. For large thermal system simulations or complex analyses, it may be more efficient to determine internal energy, enthalpy, and entropy using fundamental thermodynamic relations or curves fit to experimental data. Some of these relations are discussed in the following sections. Also, the thermodynamic relations discussed in those sections are the basis for constructing tables of thermodynamic property data. Further information on the topic may be found in references covering system modeling and thermodynamics (Howell and Buckius 1992; Stoecker 1989).
At least two intensive properties (properties independent of the quantity of substance, such as temperature, pressure, specific volume, and specific enthalpy) must be known to determine the remaining properties. If two known properties are either p , v , or T (these are relatively easy to measure and are commonly used in simulations), the third can be determined throughout the range of interest using an equation of state. Furthermore, if the specific heats at zero pressure are known, specific heat can be accurately determined from spectroscopic measurements using statistical mechanics (NASA 1971). Entropy may be considered a function of T and p , and from calculus an infinitesimal change in entropy can be written as
Likewise, a change in enthalpy can be written as
Using the Gibbs relation Tds = dh – vdp and the definition of specific heat at constant pressure, c p ≡ (∂ h /∂ T ) p , Equation (30) can be rearranged to yield
Equations (29) and (31) combine to yield (∂ s /∂ T ) p = c p / T . Then, using the Maxwell relation (∂ s /∂ p ) T = –(∂ v /∂ T ) p , Equation (29) may be rewritten as
This is an expression for an exact derivative, so it follows that
Integrating this expression at a fixed temperature yields
where c p 0 is the known zero-pressure specific heat, and dp T is used to indicate that integration is performed at a fixed temperature. The second partial derivative of specific volume with respect to temperature can be determined from the equation of state. Thus, Equation (34) can be used to determine the specific heat at any pressure.
Using Tds = dh – vdp , Equation (30) can be written as
Equations (31) and (35) may be integrated at constant pressure to obtain
Integrating the Maxwell relation (∂ s /∂ p ) T = –(∂ v /∂ T ) p gives an equation for entropy changes at a constant temperature as
Likewise, integrating Equation (33) along an isotherm yields the following equation for enthalpy changes at a constant temperature:
Internal energy can be calculated from u = h – pv . When entropy or enthalpy are known at a reference temperature T 0 and pressure p 0 , values at any temperature and pressure may be obtained by combining Equations (36) and (38) or Equations (37) and (39) .
Combinations (or variations) of Equations (36) to (39) can be incorporated directly into computer subroutines to calculate properties with improved accuracy and efficiency. However, these equations are restricted to situations where the equation of state is valid and the properties vary continuously. These restrictions are violated by a change of phase such as evaporation and condensation, which are essential processes in air-conditioning and refrigerating devices. Therefore, the Clapeyron equation is of particular value; for evaporation or condensation, it gives
If vapor pressure and liquid and vapor density data (all relatively easy measurements to obtain) are known at saturation, then changes in enthalpy and entropy can be calculated using Equation (40) .
Phase Equilibria for Multicomponent Systems
To understand phase equilibria, consider a container full of a liquid made of two components; the more volatile component is designated i and the less volatile component j ( Figure 2A ). This mixture is all liquid because the temperature is low (but not so low that a solid appears). Heat added at a constant pressure raises the mixture’s temperature, and a sufficient increase causes vapor to form, as shown in Figure 2B . If heat at constant pressure continues to be added, eventually the temperature becomes so high that only vapor remains in the container ( Figure 2C ). A temperature-concentration ( T - x ) diagram is useful for exploring details of this situation.
Figure 3 is a typical T - x diagram valid at a fixed pressure. The case shown in Figure 2A , a container full of liquid mixture with mole fraction x i, 0 at temperature T 0 , is point 0 on the T - x diagram. When heat is added, the mixture’s temperature increases. The point at which vapor begins to form is the bubble point . Starting at point 0, the first bubble forms at temperature T 1 (point 1 on the diagram). The locus of bubble points is the bubble-point curve , which provides bubble points for various liquid mole fractions x i .
Figure 2. Mixture of i and j Components in Constant-Pressure Container
When the first bubble begins to form, vapor in the bubble may not have the same mole fraction as the liquid mixture. Rather, the mole fraction of the more volatile species is higher in the vapor than in the liquid. Boiling prefers more volatile species, and the T - x diagram shows this behavior. At T l , the vapor-forming bubbles have an i mole fraction of y i,l . If heat continues to be added, this preferential boiling depletes the liquid of species i and the temperature required to continue the process increases. Again, the T- x diagram reflects this fact; at point 2 the i mole fraction in the liquid is reduced to x i ,2 and the vapor has a mole fraction of y i ,2 . The temperature required to boil the mixture is increased to T 2 . Position 2 on the T-x diagram could correspond to the physical situation shown in Figure 2B .
Figure 3. Temperature-Concentration ( T-x ) Diagram for Zeotropic Mixture
If constant-pressure heating continues, all the liquid eventually becomes vapor at temperature T 3 . The vapor at this point is shown as position 3′ in Figure 3 . At this point the i mole fraction in the vapor y i ,3 equals the starting mole fraction in the all-liquid mixture x i ,1 . This equality is required for mass and species conservation. Further addition of heat simply raises the vapor temperature. The final position 4 corresponds to the physical situation shown in Figure 2C .
Figure 4. Azeotropic Behavior Shown on T - x Diagram
Starting at position 4 in Figure 3 , heat removal leads to initial liquid formation when position 3′ (the dew point ) is reached. The locus of dew points is called the dew-point curve . Heat removal causes the liquid phase of the mixture to reverse through points 3, 2, 1, and to starting point 0. Because the composition shifts, the temperature required to boil (or condense) this mixture changes as the process proceeds. This is known as temperature glide . This mixture is therefore called zeotropic .
Most mixtures have T - x diagrams that behave in this fashion, but some have a markedly different feature. If the dew-point and bubble-point curves intersect at any point other than at their ends, the mixture exhibits azeotropic behavior at that composition. This case is shown as position a in the T - x diagram of Figure 4 . If a container of liquid with a mole fraction x a were boiled, vapor would be formed with an identical mole fraction y a . The addition of heat at constant pressure would continue with no shift in composition and no temperature glide.
Perfect azeotropic behavior is uncommon, although near-azeotropic behavior is fairly common. The azeotropic composition is pressure dependent, so operating pressures should be considered for their effect on mixture behavior. Azeotropic and near-azeotropic refrigerant mixtures are widely used. The properties of an azeotropic mixture are such that they may be conveniently treated as pure substance properties. Phase equilibria for zeotropic mixtures, however, require special treatment, using an equation-of-state approach with appropriate mixing rules or using the fugacities with the standard state method (Tassios 1993). Refrigerant and lubricant blends are a zeotropic mixture and can be treated by these methods (Martz et al. 1996a, 1996b; Thome 1995).
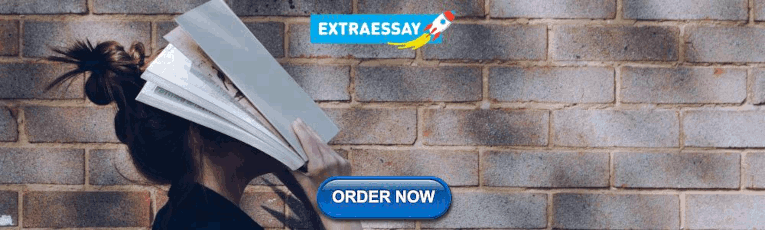
2. COMPRESSION REFRIGERATION CYCLES
2.1 carnot cycle.
The Carnot cycle, which is completely reversible, is a perfect model for a refrigeration cycle operating between two fixed temperatures, or between two fluids at different temperatures and each with infinite heat capacity. Reversible cycles have two important properties: (1) no refrigerating cycle may have a coefficient of performance higher than that for a reversible cycle operated between the same temperature limits, and (2) all reversible cycles, when operated between the same temperature limits, have the same coefficient of performance. Proof of both statements may be found in almost any textbook on elementary engineering thermodynamics.
Figure 5 shows the Carnot cycle on temperature-entropy coordinates. Heat is withdrawn at constant temperature T R from the region to be refrigerated. Heat is rejected at constant ambient temperature T 0 . The cycle is completed by an isentropic expansion and an isentropic compression. The energy transfers are given by
Thus, by Equation (16) ,
Figure 5. Carnot Refrigeration Cycle
Figure 6. Temperature-Entropy Diagram for Carnot Refrigeration Cycle of Example 1
Determine entropy change, work, and COP for the cycle shown in Figure 6 . Temperature of the refrigerated space T R is 400°R , and that of the atmosphere T 0 is 500°R . Refrigeration load is 200 Btu .
Flow of energy and its area representation in Figure 6 are
The net change of entropy of any refrigerant in any cycle is always zero. In Example 1, the change in entropy of the refrigerated space is Δ S R = −200/400 = −0.5 Btu/°R and that of the atmosphere is Δ S o = 250/ 500 = 0.5 Btu/°R . The net change in entropy of the isolated system is Δ S total = Δ S R + Δ S o = 0.
Figure 7. Carnot Vapor Compression Cycle
The Carnot cycle in Figure 7 shows a process in which heat is added and rejected at constant pressure in the two-phase region of a refrigerant. Saturated liquid at state 3 expands isentropically to the low temperature and pressure of the cycle at state d. Heat is added isothermally and isobarically by evaporating the liquid-phase refrigerant from state d to state 1. The cold saturated vapor at state 1 is compressed isentropically to the high temperature in the cycle at state b. However, the pressure at state b is below the saturation pressure corresponding to the high temperature in the cycle. The compression process is completed by an isothermal compression process from state b to state c. The cycle is completed by an isothermal and isobaric heat rejection or condensing process from state c to state 3.
Applying the energy equation for a mass of refrigerant m yields (all work and heat transfer are positive)
The net work for the cycle is
2.2 THEORETICAL SINGLE-STAGE CYCLE USING A PURE REFRIGERANT OR AZEOTROPIC MIXTURE
A system designed to approach the ideal model shown in Figure 7 is desirable. A pure refrigerant or azeotropic mixture can be used to maintain constant temperature during phase changes by maintaining constant pressure. Because of concerns such as high initial cost and increased maintenance requirements, a practical machine has one compressor instead of two and the expander (engine or turbine) is replaced by a simple expansion valve, which throttles refrigerant from high to low pressure. Figure 8 shows the theoretical single-stage cycle used as a model for actual systems.
Applying the energy equation for a mass m of refrigerant yields
Constant-enthalpy throttling assumes no heat transfer or change in potential or kinetic energy through the expansion valve.
Figure 8. Theoretical Single-Stage Vapor Compression Refrigeration Cycle
The coefficient of performance is
The theoretical compressor displacement CD (at 100% volumetric efficiency) is
which is a measure of the physical size or speed of the compressor required to handle the prescribed refrigeration load.
A theoretical single-stage cycle using R-134a as the refrigerant operates with a condensing temperature of 90°F and an evaporating temperature of 0°F . The system produces 15 tons of refrigeration. Determine the (a) thermodynamic property values at the four main state points of the cycle, (b) COP, (c) cycle refrigerating efficiency, and (d) rate of refrigerant flow.
(a) Figure 9 shows a schematic p-h diagram for the problem with numerical property data. Saturated vapor and saturated liquid properties for states 1 and 3 are obtained from the saturation table for R-134a in Chapter 30 . Properties for superheated vapor at state 2 are obtained by linear interpolation of the superheat tables for R-134a in Chapter 30 . Specific volume and specific entropy values for state 4 are obtained by determining the quality of the liquid-vapor mixture from the enthalpy.
The property data are tabulated in Table 1 .
(b) By Equation (43) ,
(c) By Equations (20) and (41) ,
(d) The mass flow of refrigerant is obtained from an energy balance on the evaporator. Thus,
Figure 9. Schematic p - h Diagram for Example 2
Table 1 Thermodynamic Property Data for Example 2
The saturation temperatures of the single-stage cycle strongly influence the magnitude of the coefficient of performance. This influence may be readily appreciated by an area analysis on a temperature-entropy ( T- s ) diagram. The area under a reversible process line on a T- s diagram is directly proportional to the thermal energy added or removed from the working fluid. This observation follows directly from the definition of entropy [see Equation (8) ].
In Figure 10 , the area representing Q o is the total area under the constant-pressure curve between states 2 and 3. The area representing the refrigerating capacity Q i is the area under the constant-pressure line connecting states 4 and 1. The net work required W net equals the difference ( Q o – Q i ), which is represented by the entire shaded area shown on Figure 10 .
Because COP = Q i / W net , the effect on the COP due to changes in evaporating temperature and condensing temperature may be observed. For example, a decrease in evaporating temperature T E significantly increases W net and slightly decreases Q i . An increase in condensing temperature T C produces the same results but with less effect on W net . Therefore, for maximum coefficient of performance, the cycle should operate at the lowest possible condensing temperature and maximum possible evaporating temperature.
Figure 10. Areas on T- s Diagram Representing Refrigerating Effect and Work Supplied for Theoretical Single-Stage Cycle
2.3 LORENZ REFRIGERATION CYCLE
The Carnot refrigeration cycle includes two assumptions that make it impractical. The heat transfer capacities of the two external fluids are assumed to be infinitely large so the external fluid temperatures remain fixed at T 0 and T R (they become infinitely large thermal reservoirs). The Carnot cycle also has no thermal resistance between the working refrigerant and external fluids in the two heat exchange processes. As a result, the refrigerant must remain fixed at T 0 in the condenser and at T R in the evaporator.
The Lorenz cycle eliminates the first restriction in the Carnot cycle by allowing the temperature of the two external fluids to vary during heat exchange. The second assumption of negligible thermal resistance between the working refrigerant and two external fluids remains. Therefore, the refrigerant temperature must change during the two heat exchange processes to equal the changing temperature of the external fluids. This cycle is completely reversible when operating between two fluids that each have a finite but constant heat capacity.
Figure 11 is a schematic of a Lorenz cycle. Note that this cycle does not operate between two fixed temperature limits. Heat is added to the refrigerant from state 4 to state 1. This process is assumed to be linear on T- s coordinates, which represents a fluid with constant heat capacity. The refrigerant temperature is increased in isentropic compression from state 1 to state 2. Process 2–3 is a heat rejection process in which the refrigerant temperature decreases linearly with heat transfer. The cycle ends with isentropic expansion between states 3 and 4.
The heat addition and heat rejection processes are parallel so the entire cycle is drawn as a parallelogram on T- s coordinates. A Carnot refrigeration cycle operating between T 0 and T R would lie between states 1, a, 3, and b; the Lorenz cycle has a smaller refrigerating effect and requires more work, but this cycle is a more practical reference when a refrigeration system operates between two single-phase fluids such as air or water.
Figure 11. Processes of Lorenz Refrigeration Cycle
The energy transfers in a Lorenz refrigeration cycle are as follows, where Δ T is the temperature change of the refrigerant during each of the two heat exchange processes.
Thus by Equation (16) ,
Determine the entropy change, work required, and COP for the Lorenz cycle shown in Figure 11 when the temperature of the refrigerated space is T R = 400°R , ambient temperature is T 0 = 500°R , Δ T of the refrigerant is 10°R , and refrigeration load is 200 Btu .
Note that the entropy change for the Lorenz cycle is larger than for the Carnot cycle when both operate between the same two temperature reservoirs and have the same capacity (see Example 1). That is, both the heat rejection and work requirement are larger for the Lorenz cycle. This difference is caused by the finite temperature difference between the working fluid in the cycle compared to the bounding temperature reservoirs. However, as discussed previously, the assumption of constant-temperature heat reservoirs is not necessarily a good representation of an actual refrigeration system because of the temperature changes that occur in the heat exchangers.
2.4 THEORETICAL SINGLE-STAGE CYCLE USING ZEOTROPIC REFRIGERANT MIXTURE
A practical method to approximate the Lorenz refrigeration cycle is to use a fluid mixture as the refrigerant and the four system components shown in Figure 8 . When the mixture is not azeotropic and the phase change occurs at constant pressure, the temperatures change during evaporation and condensation and the theoretical single-stage cycle can be shown on T-s coordinates as in Figure 12 . In comparison, Figure 10 shows the system operating with a pure simple substance or an azeotropic mixture as the refrigerant. Equations (15) , (16) , (42) , (43) , and (44) apply to this cycle and to conventional cycles with constant phase change temperatures. Equation (45) should be used as the reversible cycle COP in Equation (20) .
For zeotropic mixtures, the concept of constant saturation temperatures does not exist. For example, in the evaporator, the refrigerant enters at T 4 and exits at a higher temperature T 1 . The temperature of saturated liquid at a given pressure is the bubble point and the temperature of saturated vapor at a given pressure is called the dew point . The temperature T 3 in Figure 12 is at the bubble point at the condensing pressure and T 1 is at the dew point at the evaporating pressure.
Figure 12. Areas on T- s Diagram Representing Refrigerating Effect and Work Supplied for Theoretical Single-Stage Cycle Using Zeotropic Mixture as Refrigerant
Areas on a T- s diagram representing additional work and reduced refrigerating effect from a Lorenz cycle operating between the same two temperatures T 1 and T 3 with the same value for Δ T can be analyzed. The cycle matches the Lorenz cycle most closely when counterflow heat exchangers are used for both the condenser and evaporator.
In a cycle that has heat exchangers with finite thermal resistances and finite external fluid capacity rates, Kuehn and Gronseth (1986) showed that a cycle using a refrigerant mixture has a higher coefficient of performance than one using a simple pure substance as a refrigerant. However, the improvement in COP is usually small. Mixture performance can be improved further by reducing the heat exchangers’ thermal resistance and passing fluids through them in a counterflow arrangement.
2.5 MULTISTAGE VAPOR COMPRESSION REFRIGERATION CYCLES
Multistage or multipressure vapor compression refrigeration is used when several evaporators are needed at various temperatures, such as in a supermarket, or when evaporator temperature becomes very low. Low evaporator temperature indicates low evaporator pressure and low refrigerant density into the compressor. Two small compressors in series have a smaller displacement and usually operate more efficiently than one large compressor that covers the entire pressure range from the evaporator to the condenser. This is especially true in ammonia refrigeration systems because of the large amount of superheating that occurs during the compression process.
Thermodynamic analysis of multistage cycles is similar to analysis of single-stage cycles, except that mass flow differs through various components of the system in the former. A careful mass balance and energy balance on individual components or groups of components ensures correct application of the first law of thermodynamics. Care must also be used when performing second-law calculations. Often, the refrigerating load is comprised of more than one evaporator, so the total system capacity is the sum of the loads from all evaporators. Likewise, the total energy input is the sum of the work into all compressors. For multistage cycles, the expression for the coefficient of performance given in Equation (16) should be written as
When compressors are connected in series, vapor between stages should be cooled to bring the vapor to saturated conditions before proceeding to the next stage of compression. Intercooling usually minimizes displacement of the compressors, reduces the work requirement, and increases the cycle’s COP. If the refrigerant temperature between stages is above ambient, a simple intercooler that removes heat from the refrigerant can be used. If the temperature is below ambient, which is the usual case, the refrigerant itself must be used to cool the vapor. This is accomplished with a flash intercooler. Figure 13 shows a cycle with a flash intercooler installed.
The superheated vapor from compressor I is bubbled through saturated liquid refrigerant at the intermediate pressure of the cycle. Some of this liquid is evaporated when heat is added from the superheated refrigerant. The result is that only saturated vapor at the intermediate pressure is fed to compressor II. A common approach is to operate the intercooler at about the geometric mean of the evaporating and condensing pressures. This operating point provides the same pressure ratio and nearly equal volumetric efficiencies for the two compressors. Example 4 illustrates the thermodynamic analysis of this cycle.
Determine the thermodynamic properties of the eight state points shown in Figure 13 , mass flows, and COP of this theoretical multistage refrigeration cycle using R-134a. The saturated evaporator temperature is 0°F , the saturated condensing temperature is 90°F , and the refrigeration load is 15 tons . The saturation temperature of the refrigerant in the intercooler is 40°F , which is nearly at the geometric mean pressure of the cycle.
Thermodynamic property data are obtained from the saturation and superheat tables for R-134a in Chapter 30 . States 1, 3, 5, and 7 are obtained directly from the saturation table. State 6 is a mixture of liquid and vapor. The quality is calculated by
Similarly for state 8,
States 2 and 4 are obtained from the superheat tables by linear interpolation. The thermodynamic property data are summarized in Table 2 .
Mass flow through the lower circuit of the cycle is determined from an energy balance on the evaporator.
For the upper circuit of the cycle,
Assuming the intercooler has perfect external insulation, an energy balance on it is used to compute ṁ 3 .
Rearranging and solving for ṁ 3 ,
Table 2 Thermodynamic Property Values for Example 4
Figure 13. Schematic and Pressure-Enthalpy Diagram for Dual-Compression, Dual-Expansion Cycle of Example 4
Examples 2 and 4 have the same refrigeration load and operate with the same evaporating and condensing temperatures. The two-stage cycle in Example 4 has a higher COP and less work input than the single-stage cycle. Also, the highest refrigerant temperature leaving the compressor is about 96°F for the two-stage cycle versus about 104°F for the single-stage cycle. One of the benefits of this lower maximum temperature is better compressor lubrication and longer equipment life ( Chang et al. 2016; Wu et al. 2017 ). These differences are more pronounced for cycles operating at larger pressure ratios.
2.6 ACTUAL REFRIGERATION SYSTEMS
Actual systems operating steadily differ from the ideal cycles considered in the previous sections in many respects. Pressure drops occur everywhere in the system except in the compression process. Heat transfers between the refrigerant and its environment in all components. The actual compression process differs substantially from isentropic compression. The working fluid is not a pure substance but a mixture of refrigerant and oil. All of these deviations from a theoretical cycle cause irreversibilities in the system. Each irreversibility requires additional power into the compressor. It is useful to understand how these irreversibilities are distributed throughout a real system; this insight can be useful when design changes are contemplated or operating conditions are modified. Example 5 illustrates how the irreversibilities can be computed in a real system and how they require additional compressor power to overcome. Input data have been rounded off for ease of computation.
An air-cooled, direct-expansion, single-stage mechanical vapor-compression refrigerator uses R-22 and operates under steady conditions. A schematic of this system is shown in Figure 14 . Pressure drops occur in all piping, and heat gains or losses occur as indicated. Power input includes compressor power and the power required to operate both fans. The following performance data are obtained:
Ambient air temperature t 0 = 90°F
Refrigerated space temperature t R = 20°F
Compressor power input Ẇ comp = 3.0 hp
Condenser fan input Ẇ CF = 0.2 hp
Evaporator fan input Ẇ EF = 0.15 hp
Refrigerant pressures and temperatures are measured at the seven locations shown in Figure 14 . Table 3 lists the measured and computed thermodynamic properties of the refrigerant, neglecting the dissolved oil. A pressure-enthalpy diagram of this cycle is shown in Figure 15 and is compared with a theoretical single-stage cycle operating between the air temperatures t R and t 0 .
Compute the energy transfers to the refrigerant in each component of the system and determine the second-law irreversibility rate in each component. Show that the total irreversibility rate multiplied by the absolute ambient temperature is equal to the difference between the actual power input and the power required by a Carnot cycle operating between t R and t 0 with the same refrigerating load.
Solution: The mass flow of refrigerant is the same through all components, so it is only computed once through the evaporator. Each component in the system is analyzed sequentially, beginning with the evaporator. Equation (6) is used to perform a first-law energy balance on each component, and Equations (11) and (14) are used for the second-law analysis. Note that the temperature used in the second-law analysis is the absolute temperature.
Evaporator :
Energy balance
Suction Line :
Compressor:
Discharge Line:
Condenser :
Liquid Line :
Expansion Device :
These results are summarized in Table 4 . For the Carnot cycle,
The Carnot power requirement for the 2 ton load is
The actual power requirement for the compressor is
This result is within computational error of the measured power input to the compressor of 7635 Btu/h .
Figure 14. Schematic of Real, Direct-Expansion, Single-Stage Mechanical Vapor-Compression Refrigeration System
Figure 15. Pressure-Enthalpy Diagram of Actual System and Theoretical Single-Stage System Operating Between Same Inlet Air Temperatures t R and t 0
Table 3 Measured and Computed Thermodynamic Properties of R-22 for Example 5
The analysis in Example 5 can be applied to any actual vapor compression refrigeration system. The only required information for second-law analysis is the refrigerant thermodynamic state points and mass flow rates and the temperatures in which the system is exchanging heat. In this example, the extra compressor power required to overcome the irreversibility in each component is determined. The component with the largest loss is the compressor. This loss is due to motor inefficiency, friction losses, and irreversibilities caused by pressure drops, mixing, and heat transfer between the compressor and the surroundings. Unrestrained expansion in the expansion device is the next largest (also a large loss), but could be reduced by using an expander rather than a throttling process. An expander may be economical on large machines.
Table 4 Energy Transfers and Irreversibility Rates for Refrigeration System in Example 5
All heat transfer irreversibilities on both the refrigerant side and the air side of the condenser and evaporator are included in the analysis. Refrigerant pressure drop is also included. Air-side pressure drop irreversibilities of the two heat exchangers are not included, but these are equal to the fan power requirements because all the fan power is dissipated as heat.
An overall second-law analysis, such as in Example 5, shows the designer components with the most losses, and helps determine which components should be replaced or redesigned to improve performance. However, it does not identify the nature of the losses; this requires a more detailed second-law analysis of the actual processes in terms of fluid flow and heat transfer (Liang and Kuehn 1991). A detailed analysis shows that most irreversibilities associated with heat exchangers are due to heat transfer, whereas air-side pressure drop causes a very small loss and refrigerant pressure drop causes a negligible loss. This finding indicates that promoting refrigerant heat transfer at the expense of increasing the pressure drop often improves performance. Using a thermoeconomic technique is required to determine the cost/benefits associated with reducing component irreversibilities.
3. ABSORPTION REFRIGERATION CYCLES
An absorption cycle is a heat-activated thermal cycle. It exchanges only thermal energy with its surroundings; no appreciable mechanical energy is exchanged. Furthermore, no appreciable conversion of heat to work or work to heat occurs in the cycle.
Absorption cycles are used in applications where one or more of the exchanges of heat with the surroundings is the useful product (e.g., refrigeration, air conditioning, and heat pumping). The two great advantages of this type of cycle in comparison to other cycles with similar product are
No large, rotating mechanical equipment is required
Any source of heat can be used, including low-temperature sources (e.g., waste heat, solar heat)
All absorption cycles include at least three thermal energy exchanges with their surroundings (i.e., energy exchange at three different temperatures). The highest- and lowest-temperature heat flows are in one direction, and the mid-temperature one (or two) is in the opposite direction. In the forward cycle , the extreme (hottest and coldest) heat flows are into the cycle. This cycle is also called the heat amplifier, heat pump, conventional cycle, or Type I cycle. When extreme-temperature heat flows are out of the cycle, it is called a reverse cycle , heat transformer, temperature amplifier, temperature booster, or Type II cycle. Figure 16 illustrates both types of thermal cycles.
Figure 16. Thermal Cycles
This fundamental constraint of heat flow into or out of the cycle at three or more different temperatures establishes the first limitation on cycle performance. By the first law of thermodynamics (at steady state),
The second law requires that
with equality holding in the ideal case.
From these two laws alone (i.e., without invoking any further assumptions) it follows that, for the ideal forward cycle,
The heat ratio Q cold / Q hot is commonly called the coefficient of performance (COP) , which is the cooling realized divided by the driving heat supplied.
Heat rejected to ambient may be at two different temperatures, creating a four-temperature cycle . The ideal COP of the four-temperature cycle is also expressed by Equation (49) , with T mid signifying the entropic mean heat rejection temperature. In that case, T mid is calculated as follows:
This expression results from assigning all the entropy flow to the single temperature T mid .
The ideal COP for the four-temperature cycle requires additional assumptions, such as the relationship between the various heat quantities. Under the assumptions that Q cold = Q mid cold and Q hot = Q mid hot , the following expression results:
Absorption cycles require at least two working substances: a sorbent and a fluid refrigerant; these substances undergo phase changes. Given this constraint, many combinations are not achievable. The first result of invoking the phase change constraints is that the various heat flows assume known identities. As shown in Figure 17 , the refrigerant phase changes occur in an evaporator and a condenser, and the sorbent phase changes in an absorber and a desorber (generator). (Note that two lines connect the evaporator to the absorber and the desorber to the condenser, with one indicating vapor flow, the second carryover of liquid. In both cases, the carryover of liquid is detrimental to system performance.) For the forward absorption cycle , the highest-temperature heat is always supplied to the generator,
and the coldest heat is supplied to the evaporator:
Figure 17. Single-Effect Absorption Cycle
For the reverse absorption cycle (also called heat transformer or type II absorption cycle ), the highest-temperature heat is rejected from the absorber, and the lowest-temperature heat is rejected from the condenser.
The second result of the phase change constraint is that, for all known refrigerants and sorbents over pressure ranges of interest,
These two relations are true because the latent heat of phase change (vapor ↔ condensed phase) is relatively constant when far removed from the critical point. Thus, each heat input cannot be independently adjusted.
The ideal single-effect forward-cycle COP expression is
Equality holds only if the heat quantities at each temperature may be adjusted to specific values, which is not possible, as shown the following discussion.
The third result of invoking the phase change constraint is that only three of the four temperatures T evap , T cond , T gen , and T abs may be independently selected.
Practical liquid absorbents for absorption cycles have a significant negative deviation from behavior predicted by Raoult’s law. This has the beneficial effect of reducing the required amount of absorbent recirculation, at the expense of reduced lift ( T cond – T evap ) and increased sorption duty. In practical terms, for most absorbents,
The net result of applying these approximations and constraints to the ideal-cycle COP for the single-effect forward cycle is
In practical terms, the temperature constraint reduces the ideal COP to about 0.9, and the heat quantity constraint further reduces it to about 0.8.
Another useful result is
where T gen min is the minimum generator temperature necessary to achieve a given evaporator temperature.
Alternative approaches are available that lead to nearly the same upper limit on ideal-cycle COP. For example, one approach equates the exergy production from a “driving” portion of the cycle to the exergy consumption in a “cooling” portion of the cycle (Tozer and James 1997). This leads to the expression
Another approach derives the idealized relationship between the two temperature differences that define the cycle: the cycle lift, defined previously, and drop ( T gen – T abs ).
One important limitation of simplified analysis of absorption cycle performance is that the heat quantities are assumed to be at fixed temperatures. In most actual applications, there is some temperature change ( temperature glide ) in the various fluids supplying or acquiring heat. It is most easily described by first considering situations wherein temperature glide is not present (i.e., truly isothermal heat exchanges). Examples are condensation or boiling of pure components (e.g., supplying heat by condensing steam). Any sensible heat exchange relies on temperature glide: for example, a circulating high-temperature liquid as a heat source; cooling water or air as a heat rejection medium; or circulating chilled glycol. Even latent heat exchanges can have temperature glide, as when a multicomponent mixture undergoes phase change.
When the temperature glide of one fluid stream is small compared to the cycle lift or drop, that stream can be represented by an average temperature, and the preceding analysis remains representative. However, one advantage of absorption cycles is they can maximize benefit from low-temperature, high-glide heat sources. That ability derives from the fact that the desorption process inherently embodies temperature glide, and hence can be tailored to match the heat source glide. Similarly, absorption also embodies glide, which can be made to match the glide of the heat rejection medium.
Implications of temperature glide have been analyzed for power cycles (Ibrahim and Klein 1998), but not yet for absorption cycles.
Working fluids for absorption cycles fall into four categories, each requiring a different approach to cycle modeling and thermodynamic analysis. Liquid absorbents can be nonvolatile (i.e., vapor phase is always pure refrigerant, neglecting condensables) or volatile (i.e., vapor concentration varies, so cycle and component modeling must track both vapor and liquid concentration). Solid sorbents can be grouped by whether they are physisorbents (also known as adsorbents ), for which, as for liquid absorbents, sorbent temperature depends on both pressure and refrigerant loading (bivariance); or chemisorbents (also known as complex compounds ), for which sorbent temperature does not vary with loading, at least over small ranges.
Thermodynamic observations can predict general trends of how working fluids’ properties affect a cycle’s performance: in all four major heat exchangers (absorber, generator, condenser, and evaporator), the amount of heat exchanged is dominated by the latent heat of the refrigerant (i.e., the component that undergoes the phase change), when any phase change of the absorbent is neglected. There are two additional contributions for the absorber and generator: heat of mixing when the condensed refrigerant is mixed with the absorbent/refrigerant solution, and heating or cooling of the refrigerant/absorbent mixture during the absorption or desorption process.
Thus, the heat requirement of the generator can be estimated as the latent heat of the refrigerant plus the heat of mixing plus the heat required to heat the remaining absorbent/refrigerant solution. Both additional terms increase the generator heat requirement and thus reduce the overall cycle efficiency. Based on this observation, the ideal absorption working fluid should have high latent heat, no heat of mixing, and a low specific heat capacity.
Furthermore, heat exchanged in the solution heat exchanger is governed by the specific heat of the fluid mixture flowing through this device. Consequently, any ineffectiveness of the heat exchanger represents a loss in absorption-cycle performance that is directly related to the specific heat capacity of the fluid mixture, reinforcing the argument that a low specific heat capacity is desirable.
Finally, losses in the expansion process of the refrigerant as it enters the evaporator are also governed by the latent heat of the refrigerant (preferably large) and its specific heat capacity (preferably small), so that as little refrigerant as possible evaporates as a result of the expansion process.
Absorption working fluids should consist of refrigerants with large latent heat and absorbents with a small heat of mixing, and both absorbent and refrigerant should have as small a specific heat capacity as possible.
Heat of mixing and latent heat are determined by functional groups within the molecule; specific heat capacity is minimized when the molecule is small and of low molecular weight . Therefore, ideal working fluids should be small molecules with as many functional groups as possible. This explains why ammonia and water are still the favored refrigerants to date for absorption cycles, and why organic fluids have not yet succeeded in commercial absorption cycle applications (because of their relatively large molecular weight ).
4. ADSORPTION REFRIGERATION SYSTEMS
Adsorption is the term frequently used for solid-vapor sorption systems in which the sorbent is a solid and the sorbate a gas. Although solid-vapor sorption systems actually comprise adsorption, absorption, and chemisorption, the term adsorption is often used to contrast these systems with liquid/vapor systems, such as lithium bromide/water and ammonia/water sorption pairs.
Solid/vapor sorption media can be divided into two classes:
Bivariant systems thermodynamically behave identically to liquid/vapor systems, in which the two components [sorbent and sorbate (i.e., refrigerant)] define a thermodynamic equilibrium relation where vapor pressure, temperature, and refrigerant concentration are interrelated. These systems are commonly depicted in p - T - x or Dühring plots.
Monovariant systems thermodynamically behave like a single component substance, in which vapor pressure and temperature are interrelated via a traditional Clausius-Clapeyron relation, but are independent of refrigerant concentration within a certain refrigerant concentration range. These systems are often depicted in p - T - n or van’t Hoff plots, in which each line represents a refrigerant concentration range.
Typical examples of bivariant adsorption systems are zeolites, activated carbons, and silica gels. The most common examples of monovariant systems are metal hydrides and coordinative complex compounds (including ammoniated and hydrated complex compounds).
Practical ammonia or water refrigerant uptake concentrations for bivariant materials are typically lower than observed with their liquid vapor counterparts. Monovariant metal hydrides use hydrogen as the gaseous components. Although uptake concentrations are very low (typically in the single-digit mass percentage), the heat of reaction for metal hydrides is very high, yielding an overall energy density almost comparable to other solid/vapor sorption systems. Coordinative complex compounds can have refrigerant uptake that exceeds the capability of other solid/gas systems. The concentration range within which temperature and vapor pressure are independent of the refrigerant concentration can exceed 50%; the heat of sorption is distinctively higher than for bivariant solid/gas and liquid/vapor systems, but much lower than observed with most metal hydrides. The large refrigerant concentration range of constant vapor pressure, also referred to as the coordination sphere , lends itself to thermal energy storage applications.
The most common coordinative complex compounds are ammoniated compounds using alkali, alkali/earth, or transition metal halides (e.g., strontium chloride, calcium chloride, calcium bromide).
5. REVERSE BRAYTON CYCLE
The reverse Brayton cycle (also known as Bell–Coleman cycle or Joule cycle) achieves a refrigeration effect by moving energy in the form of heat from a thermal reservoir or a body at cold temperature to a thermal reservoir or a body at hot temperature using work input. The system deploying Reverse Brayton Cycle can be used for applications such as air drying, refrigeration, air conditioning, or heat pump. The working fluid for the reverse Brayton cycle is a non-condensable gas (air is frequently used). For the ideal cycle, the working fluid is assumed to behave as an ideal gas. Figure 18 shows the ideal reverse Brayton cycle on temperature-entropy diagram.
Figure 18. Reverse Brayton Cycle
Gas at low pressure is adiabatically compressed from state 1 to state 2. For the ideal cycle, this compression is internally reversible, making the process isentropic. Work input to the cycle is needed to achieve compression. This hot gas at high pressure then rejects energy in the form of heat to a thermal reservoir at temperature T 0 . This heat rejection occurs isobarically from state 2 to state 3. For the ideal cycle, the gas can be cooled to the temperature of the hot thermal reservoir, T 0 . The high pressure, cool gas then undergoes adiabatic expansion in an expander such as a turbine from state 3 to state 4. For the ideal cycle, this expansion is internally reversible, making the process isentropic. The cycle produces work output during expansion while substantially cooling the gas. The temperature of gas at the end of expansion is colder than the temperature of the cold thermal reservoir, T L . The cold gas at low pressure then absorbs energy in the form of heat from a thermal reservoir at temperature T L . This heat absorption occurs isobarically from state 4 to state 1. For the ideal cycle, the gas can absorb heat until its temperature equals that of the cold thermal reservoir, T L .
The energy transfers are given by
Net work input to the cycle is
For an ideal gas,
Using this relation, the cooling coefficient of performance of the ideal Brayton cycle is
Similarly, the heating coefficient of performance of the ideal Brayton cycle is
If we compare this reverse Brayton cycle with a reverse Carnot cycle operating between two thermal reservoirs at the same temperatures, T 0 and T L :
A system operating on the reverse Brayton cycle may operate as an open cycle or as a closed cycle (Dieckmann and Mallory, 1991).
In a closed cycle configuration, a pressurized gas is the working fluid.
In an open cycle configuration, the air to be cooled (or heated) is the working fluid.
In an open cycle configuration, one of the heat exchangers is eliminated in favor of direct exchange of the refrigerant (air) with the conditioned space. An open cycle configuration utilizing the reverse Brayton cycle has been used to cool aircraft cabins (Dieckmann and Mallory 1991). Although its use for climate control of commercial automobile or truck cabins is uncommon, experimental measurements on a prototype were reported by Edwards (1975) and Edwards and McDonald (1972). One of the difficulties in widespread adoption has been the relative better performance of vapor compression technology utilizing a hydrofluorocarbon refrigerant when compared to the reverse Brayton cycle (Bhatti, 1998).
Figure 19 shows the reverse Brayton cycle with an internal heat exchanger, or regenerator, on temperature-entropy coordinates. In the simple Brayton cycle, the temperature of gas leaving the high temperature heat exchanger, T 3 , is hotter than the temperature of gas leaving the low temperature heat exchanger, T 1 . The internal heat exchanger enables further cooling of the gas entering the expander. In the ideal case, the temperature of gas high pressure gas leaving the internal heat exchanger (which is the same as the temperature of gas entering the expander), T 4 , equals the temperature of low pressure gas entering the internal heat exchanger (which is same as the temperature of gas leaving the low temperature heat exchanger), T 6 . Lower the temperature of gas entering the expander, lower the temperature of gas at the end of the expansion process. This allows the cycle with the internal heat exchanger to cool the conditioned space to lower temperatures than the simple cycle.
Figure 19. Reverse Brayton Cycle with Internal Heat Exchanger
Refer to Chapter 13 of ASHRAE Handbook—HVAC Applications for more details on the application of the reverse Brayton cycle for climate control of aircraft.
Development of turbomachinery to enable practical application of the reverse Brayton cycle in air dryers, heat pumps, and refrigerators is proposed by Backman (1996). Dieckmann et al. (1979) and Toscano et al. (1982) discuss the application of the reverse Brayton cycle in a heat pump water heater. Braun et al. (2002) discuss the application of the reverse Brayton cycle in a clothes dryer. The application of the reverse Brayton cycle heat pump for domestic space heating is discussed by White (2009). NASA Goddard Flight Center sponsored the development of a cryocooler based on the reverse Brayton cycle. The development is documented by Swift (1993) and Swift (2001).
6. REVERSE STIRLING CYCLE
Refrigerators employed to achieve temperatures below −238°F are termed cryocoolers. Commonly used cryocooler designs can be one of either two types (Radebaugh, 2018):
Recuperative type and
Regenerative type.
A Stirling-cycle based cryocooler is a regenerative-type design. Multiple Stirling cryocoolers (among other designs) are utilized by NASA to cool space instruments (Ross Jr. and Boyle 2007). The working fluid for the reverse Stirling cycle is a gas with a low boiling point such as helium, hydrogen, nitrogen, or oxygen.
The reverse Stirling cycle can move energy in the form of heat from a thermal reservoir or a body at cold temperature to a thermal reservoir or a body at hot temperature using work input. For the ideal cycle, the working fluid is assumed to behave as an ideal gas. Figure 20 shows the ideal reverse Stirling cycle on temperature-entropy and pressure-volume coordinates, as well as stages in the closed Stirling cycle with directions of work and heat transfer.
Figure 20. Reverse Stirling Cycle (Beta Configuration)
At state 1, the piston is at its bottom dead center. The piston is forced from its bottom dead center to its top dead center in an iso-thermal process, thus compressing the gas at high temperature, T 0 , from low pressure to high pressure. To maintain a constant temperature of gas, the gas rejects energy in the form of heat through the walls of the cylinder to the source at temperature T 0 . At state 2, the piston is at its top dead center. The displacer then moves from its top dead center to its bottom dead center. The gas at high pressure and hot temperature, T 0 , flows through the regenerator to the chamber exposed to the sink at temperature T L . As gas flows through the regenerator, it rejects energy in the form of heat to the regenerator. In the ideal case, its temperature reduces from T 0 to T L . Since the piston does not move, the process is isochoric. At state 3, the piston is still at its top dead center. Gas at high pressure and low temperature accepts heat from the cold thermal reservoir at T L . To maintain a constant temperature of gas, the gas expands from high pressure to low pressure and the piston moves from its top dead center to its bottom dead center. Work is done by the cycle. At state 4, the piston is at its bottom dead center. The displacer moves from its bottom dead center to its top dead center, thus forcing gas away from the chamber exposed to the cold thermal reservoir at temperature T L . As gas flows through the regenerator, it accepts energy in the form of heat from the regenerator. In the ideal case, its temperature increases from T L to T 0 . Since the piston does not move, the process is isochoric.
For the reverse Stirling cycle, the only external heat transfers take place with thermal reservoirs at temperatures T 0 and T L . If we compare this reverse Stirling cycle with a reverse Carnot cycle operating between two thermal reservoirs at the same temperatures, T 0 and T L :
Stirling engines (or coolers) can be constructed in the following arrangements (Briggs 2016):
Alpha arrangement; two pistons (compressor and displacer) in two collinear cylinders with the regenerator between the two cylinders. The hot thermal reservoir is in contact with one cylinder and the cold thermal reservoir is in contact with the other cylinder. Gas can flow between the two cylinders.
Beta arrangement; two pistons (compressor and displacer) in a single cylinder. Both thermal reservoirs and the regenerator are in contact with this cylinder.
Gamma arrangement; two pistons (compressor and displacer) in two separate cylinder where both heat exchangers and the regenerator are in contact with the cylinder in which the displacer reciprocates. The compressor piston reciprocates in the other cylinder. Gas can flow between the two cylinders.
In all arrangements except Beta arrangement, the two pistons are mechanically coupled. A Beta arrangement where the two pistons are not mechanically coupled is termed a free-piston configuration. Walker et al. (1982) present an overview of fundamentals of the Stirling cycle heat pump.
Refer to Chapter 47 of the 2018 ASHRAE Handbook—Refrigeration for more details on the application of the reverse Stirling cycle in cryocoolers.
The application of a Stirling cycle using helium as the working fluid in a domestic refrigerator is discussed by Oguz and Ozkadi (2002). Berchowitz et al. (1999) report experimental measurements for a 40 W Stirling cooler utilizing helium as the working fluid. Berchowitz et al. (2008) propose the application of a CO 2 Stirling heat pump in residential HVAC where the heat pump is driven by a heat engine. Hermes and Barbosa (2011) compare the thermodynamic performance of small portable coolers that employ different cooling technologies: thermoelectric (capacity about 15 W), Stirling cycle (capacity about 20 W), and vapor compression (capacity about 25 W).
7. SYMBOLS
Alefeld, G., and R. Radermacher. 1994. Heat conversion system s. CRC Press, Boca Raton.
ASHRAE. 2010. Designation and safety classification of refrigerants. ANSI/ASHRAE Standard 34-2010.
Backman, J. (1996). On the reversed Brayton cycle with high speed machinery [Doctoral dissertation]. https://www.osti.gov/etdeweb/biblio/464548 .
Benedict, M. 1937. Pressure, volume, temperature properties of nitrogen at high density, I and II. Journal of American Chemists Society 59(11): 2224-2233 and 2233-2242.
Benedict, M., G.B. Webb, and L.C. Rubin. 1940. An empirical equation for thermodynamic properties of light hydrocarbons and their mixtures. Journal of Chemistry and Physics 4:334.
Berchowitz, D. M. 1992. Free-piston Stirling coolers. In Proceedings of the 4th International Refrigeration and Air Conditioning Conference , West Lafayette, IN. Purdue University, West Lafayette, IN. https://docs.lib.purdue.edu/iracc/171/ .
Berchowitz, D. M., Janssen, M., & Pellizzari, R. O. 2008. CO 2 Stirling heat pump for residential use. In Proceedings of the 19th International Refrigeration and Air Conditioning Conference, July 14-17, West Lafayette, IN. Purdue University, West Lafayette, IN. https://docs.lib.purdue.edu/iracc/933/ .
Berchowitz, D.M., J. McEntee, and S. Welty. 1999. Design and testing of a 40 W free-piston Stirling cycle cooling unit. In Proceedings of the 20th In-ternational Congress of Refrigeration, Sydney, Australia, September 19-24, 1999. International Institute of Refrigeration, Paris, France.
Bhatti, M. 1998. “Open Air Cycle Air Conditioning System for Motor Vehicles,” SAE Technical Paper 980289, https://doi.org/10.4271/980289 .
Braun, J., P. Bansal, and E. Groll. 2002. Energy efficiency analysis of air cycle heat pump dryers. International Journal of Refrigeration, 25(7), 954-965. https://doi.org/10.1016/s0140-7007(01)00097-4 .
Briggs, M.H. 2016. Improving free-piston Stirling engine power density (NASA/TM-2016-219144). NASA Glenn Research Center; Cleveland, OH.
Briggs, S.W. 1971. Concurrent, crosscurrent, and countercurrent absorption in ammonia-water absorption refrigeration. ASHRAE Transactions 77(1):171.
Chang, M., Park, J., Choi, Y. et al. 2016. Reliability evaluation of scroll compressor for system air conditioner. J Mech Sci Technol 30, 4459-4463. https://doi.org/10.1007/s12206-016-0912-0 .
Cooper, H.W., and J.C. Goldfrank. 1967. B-W-R constants and new correlations. Hydrocarbon Processing 46(12):141.
Dieckmann, J.T., A.J. Erickson, A.C. Harvey, and W.M. Toscano. (1979). Research and development of an air-cycle heat-pump water heater (ORNL/SUB-7226/1). US Department of Energy, Division of Buildings and Community Systems. https://doi.org/10.2172/5749191 .
Dieckmann, J., and D. Mallory. 1991. Study of long term options for electric vehicle air conditioning (EGG-EP-9831). US Department of Energy, Washington, DC. https://doi.org/10.2172/10152952 .
Edwards, T. 1975. “The Rovac Automotive Air Conditioning System.” SAE Technical Paper 750403. https://doi.org/10.4271/750403 .
Edwards, T. and A. McDonald. 1972. ROVACS: A New Rotary-Vane Air-Cycle Air-Conditioning and Refrigeration System. SAE Technical Paper 720079, https://doi.org/10.4271/720079 .
Erickson, D.C., and M. Rane. 1994. Advanced absorption cycle: Vapor exchange GAX. Proceedings of the International Absorption Heat Pump Conference , Chicago.
Feuerecker, G., J. Scharfe, I. Greiter, C. Frank, and G. Alefeld. 1993. Measurement of thermophysical properties of aqueous LiBr solutions at high temperatures and concentrations. Proceedings of the International Absorption Heat Pump Conference , New Orleans, AES-30, pp. 493-499. American Society of Mechanical Engineers, New York.
Hanna, W.T., et al. 1995. Pinch-point analysis: An aid to understanding the GAX absorption cycle. ASHRAE Technical Data Bulletin 11(2).
Hellman, H.-M., and G. Grossman. 1996. Improved property data correlations of absorption fluids for computer simulation of heat pump cycles. ASHRAE Transactions 102(1):980-997.
Hermes, C.J., and J.R. Barbosa, Jr. 2011. Thermodynamic comparison of thermoelectric, Stirling, and vapor compression portable coolers. In Proceedings of the 23rd IIR International Congress of Refrigeration: Prague, Czech Republic, August 21-26, 2011. International Institute of Refrigeration, Paris, France.
Herold, K.E., et al. 1991. The branched GAX absorption heat pump cycle. Proceedings of Absorption Heat Pump Conference , Tokyo.
Hirschfelder, J.O., et al. 1958. Generalized equation of state for gases and liquids. Industrial and Engineering Chemistry 50:375.
Holldorff, G. 1979. Revisions up absorption refrigeration efficiency. Hydrocarbon Processing 58(7):149.
Howell, J.R., and R.O. Buckius. 1992. Fundamentals of engineering thermodynamics , 2nd ed. McGraw-Hill, New York.
Hust, J.G., and R.D. McCarty. 1967. Curve-fitting techniques and applications to thermodynamics. Cryogenics 8:200.
Hust, J.G., and R.B. Stewart. 1966. Thermodynamic property computations for system analysis. ASHRAE Journal 2:64.
Ibrahim, O.M., and S.A. Klein. 1993. Thermodynamic properties of ammonia-water mixtures. ASHRAE Transactions 99(1):1495-1502.
Ibrahim, O.M., and S.A. Klein. 1998. The maximum power cycle: A model for new cycles and new working fluids. Proceedings of the ASME Advanced Energy Systems Division , AES vol. 117. American Society of Mechanical Engineers. New York.
IIR. 1994. R123—Thermodynamic and physical properties . NH 3 −H 2 O. International Institute of Refrigeration, Paris.
Kuehn, T.H., and R.E. Gronseth. 1986. The effect of a nonazeotropic binary refrigerant mixture on the performance of a single stage refrigeration cycle. Proceedings of the International Institute of Refrigeration Conference , Purdue University, p. 119.
Langeliers, J., P. Sarkisian, and U. Rockenfeller. 2003. Vapor pressure and specific heat of Li-Br H 2 O at high temperature. ASHRAE Transactions 109(1):423-427.
Liang, H., and T.H. Kuehn. 1991. Irreversibility analysis of a water to water mechanical compression heat pump. Energy 16(6):883.
Macriss, R.A. 1968. Physical properties of modified LiBr solutions. AGA Symposium on Absorption Air-Conditioning Systems, February.
Macriss, R.A., and T.S. Zawacki. 1989. Absorption fluid data survey: 1989 update. Oak Ridge National Laboratory, Oak Ridge, TN. Report ORNL/Sub84-47989/4.
Macriss, R.A., J.M. Gutraj, and T.S. Zawacki. 1988. Absorption fluids data survey: Final report on worldwide data. Institute of Gas Technology, Chicago. web.ornl.gov/info/reports/1988/3445603155476.pdf
Martin, J.J., and Y. Hou. 1955. Development of an equation of state for gases. AIChE Journal 1:142.
Martz, W.L., C.M. Burton, and A.M. Jacobi. 1996a. Liquid-vapor equilibria for R-22, R-134a, R-125, and R-32/125 with a polyol ester lubricant: Measurements and departure from ideality. ASHRAE Transactions 102(1):367-374.
Martz, W.L., C.M. Burton, and A.M. Jacobi. 1996b. Local composition modeling of the thermodynamic properties of refrigerant and oil mixtures. International Journal of Refrigeration 19(1):25-33.
Melinder, A. 1998. Thermophysical properties of liquid secondary refrigerants. Engineering Licentiate Thesis, Department of Energy Technology, The Royal Institute of Technology, Stockholm.
Modahl, R.J., and F.C. Hayes. 1988. Evaluation of commercial advanced absorption heat pump. Proceedings of the 2nd DOE/ORNL Heat Pump Conference , Washington, D.C.
NASA. 1971. Computer program for calculation of complex chemical equilibrium composition, rocket performance, incident and reflected shocks and Chapman-Jouguet detonations. SP-273. U.S. Government Printing Office, Washington, D.C.
Oguz, E., and F. Ozkadi. 2002. Experimental investigation of a Stirling cycle cooled domestic refrigerator. In Proceedings of the 9th International Refrigeration and Air Conditioning Conference, West Lafayette, IN. Purdue University, West Lafayette, IN. https://docs.lib.purdue.edu/iracc/623/ .
Phillips, B. 1976. Absorption cycles for air-cooled solar air conditioning. ASHRAE Transactions 82(1):966.
Radebaugh, R. (2018). Cryocoolers. Cryogenic Technology Resources. Retrieved March 23, 2020, from https://trc.nist.gov/cryogenics/cryocoolers.html .
Rockenfeller, U., and L.D. Kirol. 1989. Industrial heat pumps using complex compound working media. ASME Winter Annual Meeting, December.
Rockenfeller, U., and L.D. Kirol. 1996. Commercialization of complex-compound refrigeration modules. International Absorption Heat Pump Conference, September 1996, Montréal, Québec, Canada.
Rockenfeller, U., P. Sarkisian, and L.D. Kirol. 1992. Coordinative complex compounds for efficient storage of polar refrigerants and gases. SAE Technical Paper 929275. Intersociety Energy Conversion Engineering Conference, August, San Diego, CA. SAE International, Warrendale, PA. papers.sae.org/929275/ .
Rockenfeller, U., L.D. Kirol, and K. Khalili. 1993. High-temperature waste heat driven cooling using sorption media. SAE Technical Paper 932113. 23rd International Conference on Environmental Systems, July, Colorado Springs, CO. SAE International, Warrendale, PA. papers.sae.org/932113/ .
Ross, Jr., R.G., and R.F. Boyle. 2007. An overview of NASA space cryocooler programs-2006. In 14th International Cryocooler Conference, Annapolis, MD, June 14-16, 2006 (pp. 1-10). ICC Press, Boulder, CO. https://cryocooler.org/Cryocoolers-14 .
Stewart, R.B., R.T. Jacobsen, and S.G. Penoncello. 1986. ASHRAE thermodynamic properties of refrigerants.
Stoecker, W.F. 1989. Design of thermal systems , 3rd ed. McGraw-Hill, New York.
Strobridge, T.R. 1962. The thermodynamic properties of nitrogen from 64 to 300 K, between 0.1 and 200 atmospheres. National Bureau of Standards Technical Note 129.
Swift, W.L. 1993. Preliminary design for a reverse Brayton cycle cryogenic cooler (NASA-CR-189341, NAS 1.26:189341, CREARE-TN-508). NASA, United States.
Swift, W. L. 2001. A low temperature, reverse Brayton cryocooler (Creare-52). NASA, United States.
Tassios, D.P. 1993. Applied chemical engineering thermodynamics . Springer-Verlag, New York.
Thome, J.R. 1995. Comprehensive thermodynamic approach to modeling refrigerant-lubricant oil mixtures. International Journal of Heating, Ventilating, Air Conditioning and Refrigeration Research (now Science and Technology for the Built Environment ) 1(2):110.
Tillner-Roth, R., and D.G. Friend. 1998a. Survey and assessment of available measurements on thermodynamic properties of the mixture {water + ammonia}. Journal of Physical and Chemical Reference Data 27(1)S: 45-61.
Tillner-Roth, R., and D.G. Friend. 1998b. A Helmholtz free energy formulation of the thermodynamic properties of the mixture {water + ammonia}. Journal of Physical and Chemical Reference Data 27(1)S:63-96.
Toscano, W.M., and A.C. Harvey. 1982. Research and development of an air-cycle heat pump water heater. In ASHRAE Transactions, 1982 Winter Conference, Houston, TX, Vol. 88, Part 1. American Society of Heating, Refrigerating and Air-Conditioning Engineers, Atlanta, GA.
Tozer, R.M., and R.W. James. 1997. Fundamental thermodynamics of ideal absorption cycles. International Journal of Refrigeration 20(2):123-135.
Walker, G., Fauvel, R., Gustafson, R., & van Bentham, J. (1982). Stirling engine heat pumps. International Journal of Refrigeration, 5(2), 91-97. https://doi.org/10.1016/0140-7007(82)90083-4 .
White, A. 2009. Thermodynamic analysis of the reverse Joule-Brayton cycle heat pump for domestic heating. Applied Energy , 86(11), 2443-2450. https://doi.org/10.1016/j.apenergy.2009.02.012 .
Wu, X., Z. Xing, Z. He, X. Wang, and W. Chen. 2017. Effects of lubricating oil on the performance of a semi-hermetic twin screw refrigeration compressor. Applied Thermal Engineering , 112, 340-351. https://doi.org/10.1016/j.applthermaleng.2016.10.038 .
BIBLIOGRAPHY
Bogart, M. 1981. Ammonia absorption refrigeration in industrial processes . Gulf Publishing Co., Houston.
Herold, K.E., R. Radermacher, and S.A. Klein. 1996. Absorption chillers and heat pumps. CRC Press, Boca Raton.
Jain, P.C., and G.K. Gable. 1971. Equilibrium property data for aqua-ammonia mixture. ASHRAE Transactions 77(1):149.
Moran, M.J., and H. Shapiro. 1995. Fundamentals of engineering thermodynamics, 3rd ed. John Wiley & Sons, New York.
Pátek, J., and J. Klomfar. 1995. Simple functions for fast calculations of selected thermodynamic properties of the ammonia-water system. International Journal of Refrigeration 18(4):228-234.
Stoecker, W.F., and J.W. Jones. 1982. Refrigeration and air conditioning , 2nd ed. McGraw-Hill, New York.
Van Wylen, C.J., and R.E. Sonntag. 1985. Fundamentals of classical thermodynamics , 3rd ed. John Wiley & Sons, New York.
Wark, Jr., K., and D.E. Richards. 1999. Thermodynamics (6th ed.). WCB McGraw-Hill, Boston.
Zawacki, T.S. 1999. Effect of ammonia-water mixture database on cycle calculations. Proceedings of the International Sorption Heat Pump Conference , Munich.
The preparation of the third and fourth parts is assigned to TC 8.3, Absorption and Heat Operated Machines. The remaining parts of this chapter are assigned to TC 1.1, Thermodynamics and Psychrometrics.
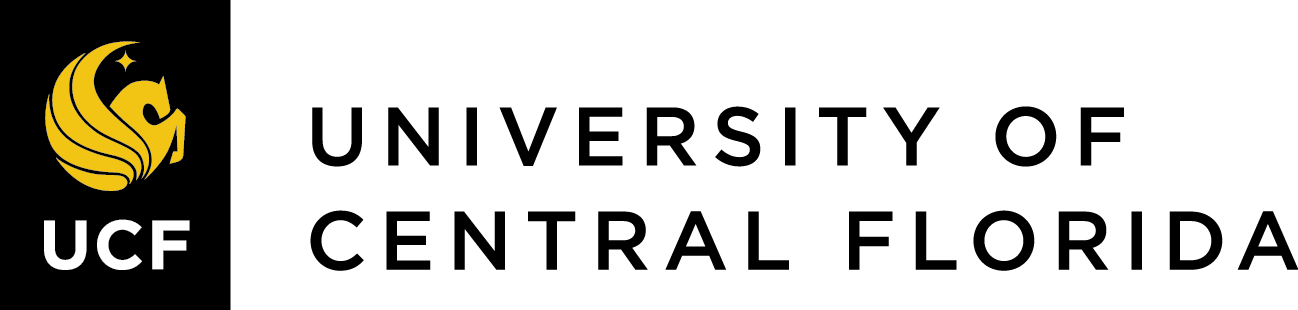
Chapter 15 Thermodynamics
15.5 Applications of Thermodynamics: Heat Pumps and Refrigerators
- Describe the use of heat engines in heat pumps and refrigerators.
- Demonstrate how a heat pump works to warm an interior space.
- Explain the differences between heat pumps and refrigerators.
- Calculate a heat pump’s coefficient of performance.
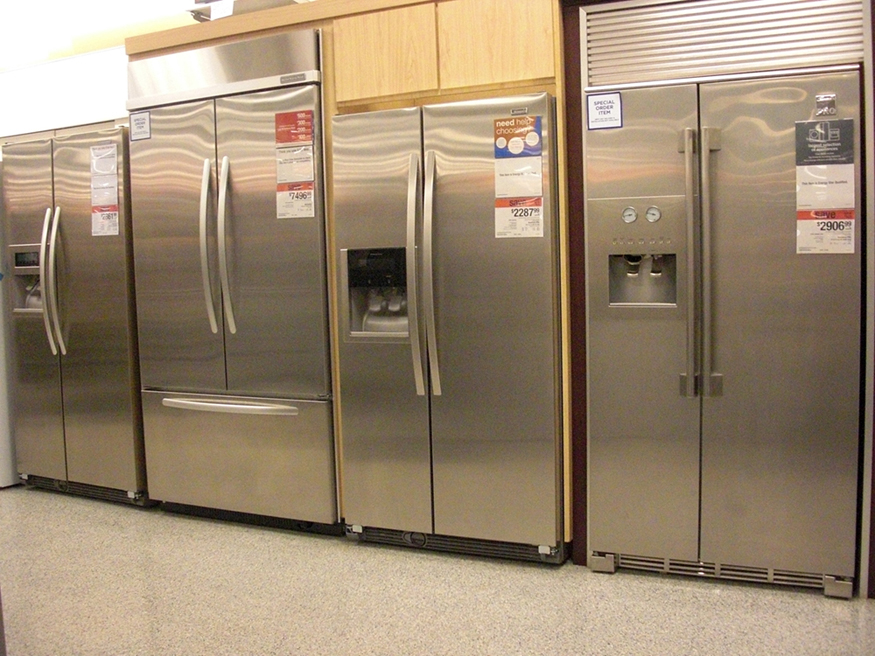
Friction and other irreversible processes reduce heat engine efficiency, but they do not benefit the operation of a heat pump—instead, they reduce the work input by converting part of it to heat transfer back into the cold reservoir before it gets into the heat pump.
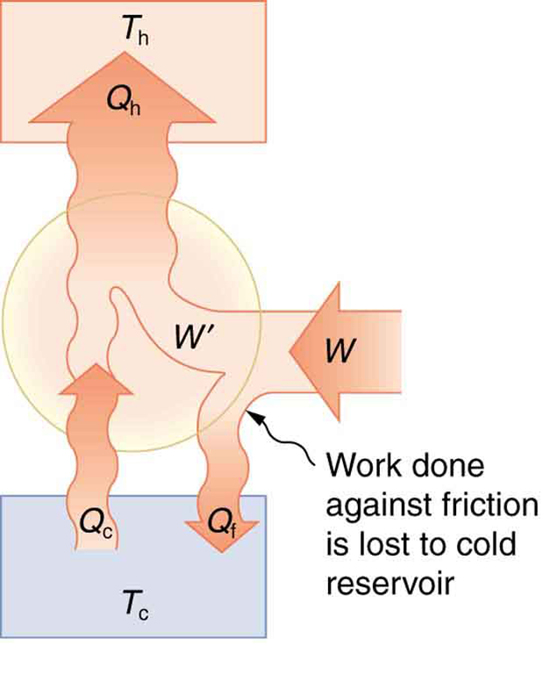
Example 1: The Best COP hp of a Heat Pump for Home Use

Carnot efficiency in terms of absolute temperature is given by :

Thus, from the discussion above,

This result means that the heat transfer by the heat pump is 5.30 times as much as the work put into it. It would cost 5.30 times as much for the same heat transfer by an electric room heater as it does for that produced by this heat pump. This is not a violation of conservation of energy. Cold ambient air provides 4.3 J per 1 J of work from the electrical outlet.
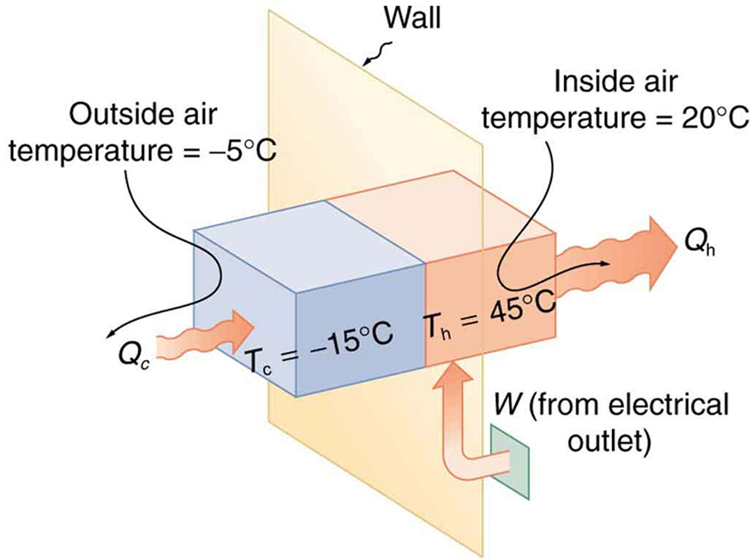
Air Conditioners and Refrigerators

PROBLEM-SOLVING STRATEGIES FOR THERMODYNAMICS
- Examine the situation to determine whether heat, work, or internal energy are involved. Look for any system where the primary methods of transferring energy are heat and work. Heat engines, heat pumps, refrigerators, and air conditioners are examples of such systems.
- Identify the system of interest and draw a labeled diagram of the system showing energy flow.
- Identify exactly what needs to be determined in the problem (identify the unknowns). A written list is useful. Maximum efficiency means a Carnot engine is involved. Efficiency is not the same as the coefficient of performance.
- Make a list of what is given or can be inferred from the problem as stated (identify the knowns). Be sure to distinguish heat transfer into a system from heat transfer out of the system, as well as work input from work output. In many situations, it is useful to determine the type of process, such as isothermal or adiabatic.
- Solve the appropriate equation for the quantity to be determined (the unknown).
- Substitute the known quantities along with their units into the appropriate equation and obtain numerical solutions complete with units.
- Check the answer to see if it is reasonable: Does it make sense? For example, efficiency is always less than 1, whereas coefficients of performance are greater than 1.
Section Summary
- An artifact of the second law of thermodynamics is the ability to heat an interior space using a heat pump. Heat pumps compress cold ambient air and, in so doing, heat it to room temperature without violation of conservation principles.
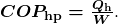
- A refrigerator is a heat pump; it takes warm ambient air and expands it to chill it.
Conceptual Questions
1: Explain why heat pumps do not work as well in very cold climates as they do in milder ones. Is the same true of refrigerators?
2: In some Northern European nations, homes are being built without heating systems of any type. They are very well insulated and are kept warm by the body heat of the residents. However, when the residents are not at home, it is still warm in these houses. What is a possible explanation?

4: Grocery store managers contend that there is less total energy consumption in the summer if the store is kept at a low temperature. Make arguments to support or refute this claim, taking into account that there are numerous refrigerators and freezers in the store.
5: Can you cool a kitchen by leaving the refrigerator door open?
Problems & Exercises

(b) 40 cents
(c) This cost seems quite realistic; it says that running an air conditioner all day would cost $9.59 (if it ran continuously).
College Physics Copyright © August 22, 2016 by OpenStax is licensed under a Creative Commons Attribution 4.0 International License , except where otherwise noted.
Share This Book
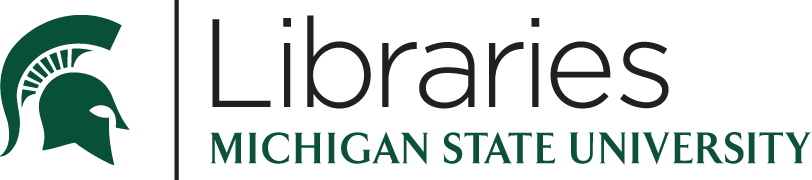
Want to create or adapt books like this? Learn more about how Pressbooks supports open publishing practices.
76 Applications of Thermodynamics: Heat Pumps and Refrigerators
[latexpage]
Learning Objectives
- Describe the use of heat engines in heat pumps and refrigerators.
- Demonstrate how a heat pump works to warm an interior space.
- Explain the differences between heat pumps and refrigerators.
- Calculate a heat pump’s coefficient of performance.
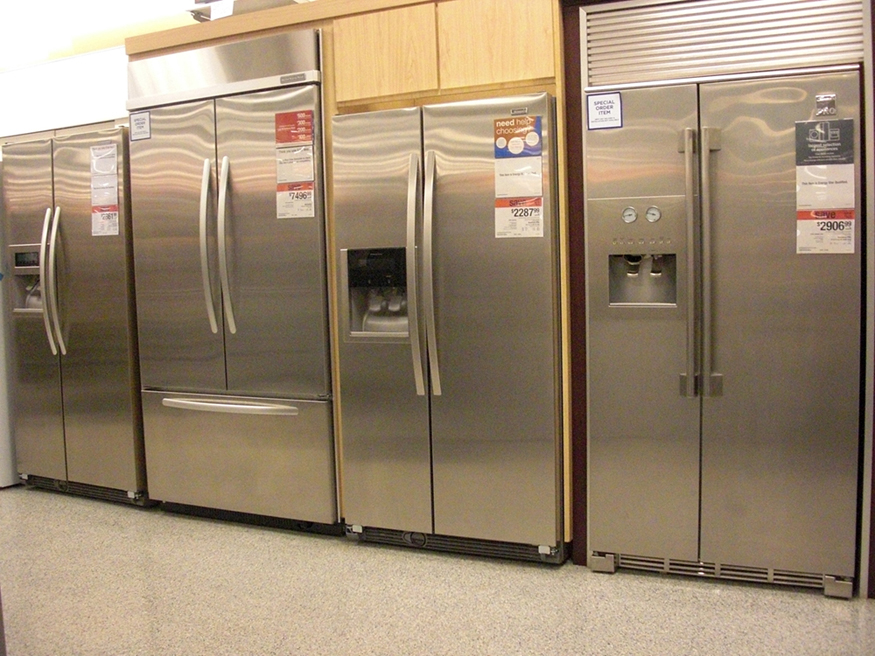
Heat pumps, air conditioners, and refrigerators utilize heat transfer from cold to hot. They are heat engines run backward. We say backward, rather than reverse, because except for Carnot engines, all heat engines, though they can be run backward, cannot truly be reversed. Heat transfer occurs from a cold reservoir \({Q}_{\text{c}}\) and into a hot one. This requires work input \(W\), which is also converted to heat transfer. Thus the heat transfer to the hot reservoir is \({Q}_{\text{h}}={Q}_{\text{c}}+W\). (Note that \({Q}_{\text{h}}\), \({Q}_{\text{c}}\), and \(W\) are positive, with their directions indicated on schematics rather than by sign.) A heat pump’s mission is for heat transfer \({Q}_{\text{h}}\) to occur into a warm environment, such as a home in the winter. The mission of air conditioners and refrigerators is for heat transfer \({Q}_{\text{c}}\) to occur from a cool environment, such as chilling a room or keeping food at lower temperatures than the environment. (Actually, a heat pump can be used both to heat and cool a space. It is essentially an air conditioner and a heating unit all in one. In this section we will concentrate on its heating mode.)
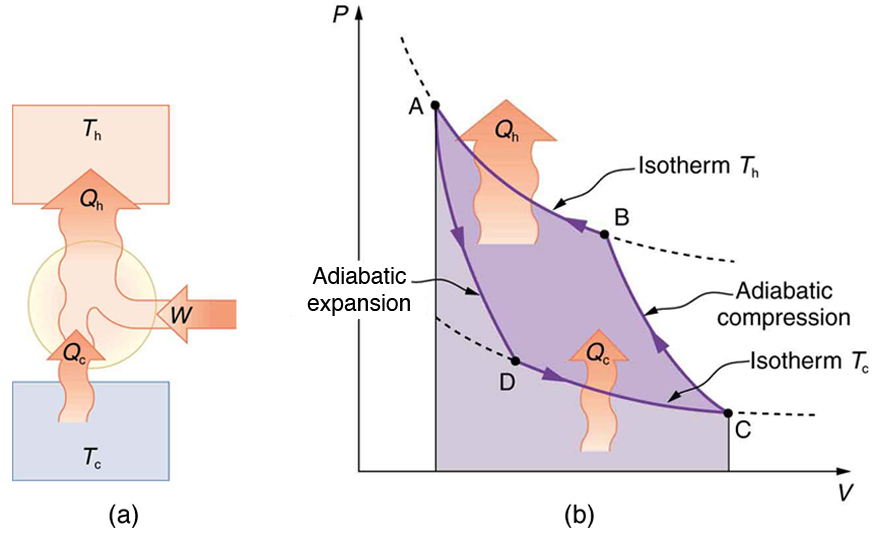
The great advantage of using a heat pump to keep your home warm, rather than just burning fuel, is that a heat pump supplies \({Q}_{\text{h}}={Q}_{\text{c}}+W\). Heat transfer is from the outside air, even at a temperature below freezing, to the indoor space. You only pay for \(W\), and you get an additional heat transfer of \({Q}_{\text{c}}\) from the outside at no cost; in many cases, at least twice as much energy is transferred to the heated space as is used to run the heat pump. When you burn fuel to keep warm, you pay for all of it. The disadvantage is that the work input (required by the second law of thermodynamics) is sometimes more expensive than simply burning fuel, especially if the work is done by electrical energy.
The basic components of a heat pump in its heating mode are shown in (Figure) . A working fluid such as a non-CFC refrigerant is used. In the outdoor coils (the evaporator), heat transfer \({Q}_{\text{c}}\) occurs to the working fluid from the cold outdoor air, turning it into a gas.
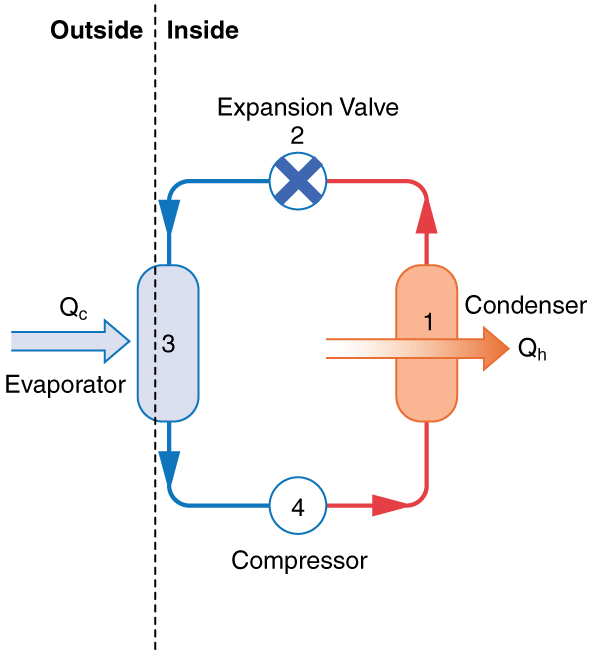
The electrically driven compressor (work input \(W\)) raises the temperature and pressure of the gas and forces it into the condenser coils that are inside the heated space. Because the temperature of the gas is higher than the temperature inside the room, heat transfer to the room occurs and the gas condenses to a liquid. The liquid then flows back through a pressure-reducing valve to the outdoor evaporator coils, being cooled through expansion. (In a cooling cycle, the evaporator and condenser coils exchange roles and the flow direction of the fluid is reversed.)
The quality of a heat pump is judged by how much heat transfer \({Q}_{\text{h}}\) occurs into the warm space compared with how much work input \(W\) is required. In the spirit of taking the ratio of what you get to what you spend, we define a heat pump’s coefficient of performance (\({\text{COP}}_{\text{hp}}\)) to be
Since the efficiency of a heat engine is \(\text{Eff}=W/{Q}_{\text{h}}\), we see that \({\text{COP}}_{\text{hp}}=1/\text{Eff}\), an important and interesting fact. First, since the efficiency of any heat engine is less than 1, it means that \({\text{COP}}_{\text{hp}}\) is always greater than 1—that is, a heat pump always has more heat transfer \({Q}_{\text{h}}\) than work put into it. Second, it means that heat pumps work best when temperature differences are small. The efficiency of a perfect, or Carnot, engine is \({\text{Eff}}_{\text{C}}=1-\left({T}_{\text{c}}/{T}_{\text{h}}\right)\); thus, the smaller the temperature difference, the smaller the efficiency and the greater the \({\text{COP}}_{\text{hp}}\) (because \({\text{COP}}_{\text{hp}}=1/\text{Eff}\)). In other words, heat pumps do not work as well in very cold climates as they do in more moderate climates.
Friction and other irreversible processes reduce heat engine efficiency, but they do not benefit the operation of a heat pump—instead, they reduce the work input by converting part of it to heat transfer back into the cold reservoir before it gets into the heat pump.
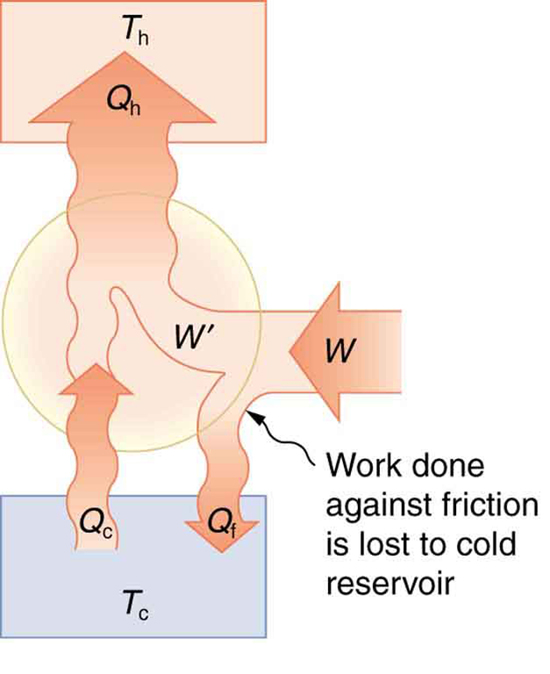
A heat pump used to warm a home must employ a cycle that produces a working fluid at temperatures greater than typical indoor temperature so that heat transfer to the inside can take place. Similarly, it must produce a working fluid at temperatures that are colder than the outdoor temperature so that heat transfer occurs from outside. Its hot and cold reservoir temperatures therefore cannot be too close, placing a limit on its \({\text{COP}}_{\text{hp}}\). (See (Figure) .) What is the best coefficient of performance possible for such a heat pump, if it has a hot reservoir temperature of \(\text{45}\text{.}0\text{º}\text{C}\) and a cold reservoir temperature of \(-\text{15}\text{.}0\text{º}\text{C}\)?
A Carnot engine reversed will give the best possible performance as a heat pump. As noted above, \({\text{COP}}_{\text{hp}}=1/\text{Eff}\), so that we need to first calculate the Carnot efficiency to solve this problem.
Carnot efficiency in terms of absolute temperature is given by :
The temperatures in kelvins are \({T}_{\text{h}}=\text{318 K}\) and \({T}_{\text{c}}=\text{258 K}\), so that
Thus, from the discussion above,
This result means that the heat transfer by the heat pump is 5.30 times as much as the work put into it. It would cost 5.30 times as much for the same heat transfer by an electric room heater as it does for that produced by this heat pump. This is not a violation of conservation of energy. Cold ambient air provides 4.3 J per 1 J of work from the electrical outlet.
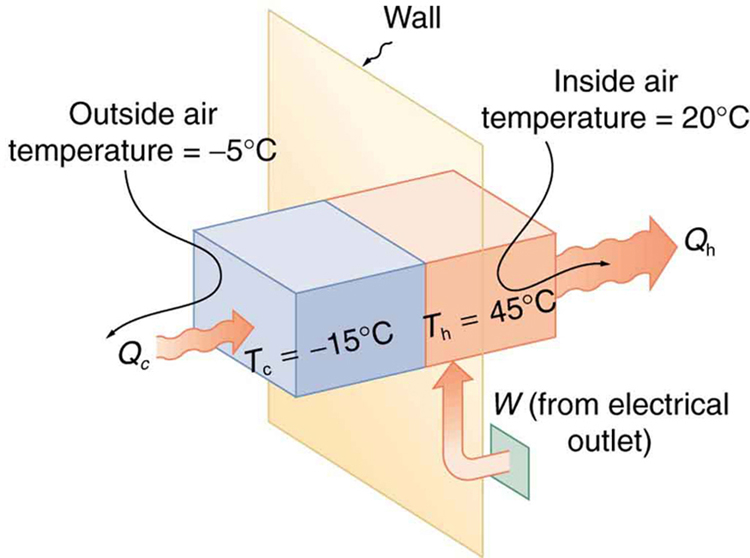
Real heat pumps do not perform quite as well as the ideal one in the previous example; their values of \({\text{COP}}_{\text{hp}}\) range from about 2 to 4. This range means that the heat transfer \({Q}_{\text{h}}\) from the heat pumps is 2 to 4 times as great as the work \(W\) put into them. Their economical feasibility is still limited, however, since \(W\) is usually supplied by electrical energy that costs more per joule than heat transfer by burning fuels like natural gas. Furthermore, the initial cost of a heat pump is greater than that of many furnaces, so that a heat pump must last longer for its cost to be recovered. Heat pumps are most likely to be economically superior where winter temperatures are mild, electricity is relatively cheap, and other fuels are relatively expensive. Also, since they can cool as well as heat a space, they have advantages where cooling in summer months is also desired. Thus some of the best locations for heat pumps are in warm summer climates with cool winters. (Figure) shows a heat pump, called a “ reverse cycle” or “ split-system cooler” in some countries.
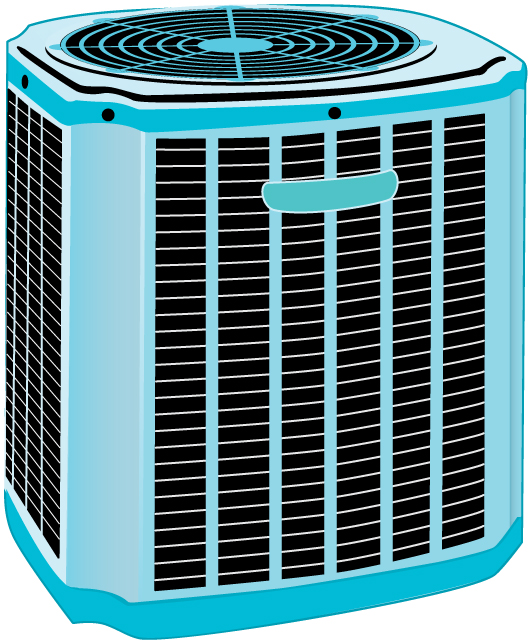
Air Conditioners and Refrigerators
Air conditioners and refrigerators are designed to cool something down in a warm environment. As with heat pumps, work input is required for heat transfer from cold to hot, and this is expensive. The quality of air conditioners and refrigerators is judged by how much heat transfer \({Q}_{\text{c}}\) occurs from a cold environment compared with how much work input \(W\) is required. What is considered the benefit in a heat pump is considered waste heat in a refrigerator. We thus define the coefficient of performance \({\text{(COP}}_{\text{ref}}\text{)}\) of an air conditioner or refrigerator to be
Noting again that \({Q}_{\text{h}}={Q}_{\text{c}}+W\), we can see that an air conditioner will have a lower coefficient of performance than a heat pump, because \({\text{COP}}_{\text{hp}}={Q}_{\text{h}}/W\) and \({Q}_{\text{h}}\) is greater than \({Q}_{\text{c}}\). In this module’s Problems and Exercises, you will show that
for a heat engine used as either an air conditioner or a heat pump operating between the same two temperatures. Real air conditioners and refrigerators typically do remarkably well, having values of \({\text{COP}}_{\text{ref}}\) ranging from 2 to 6. These numbers are better than the \({\text{COP}}_{\text{hp}}\) values for the heat pumps mentioned above, because the temperature differences are smaller, but they are less than those for Carnot engines operating between the same two temperatures.
A type of \(\text{COP}\) rating system called the “energy efficiency rating” (\(\text{EER}\) ) has been developed. This rating is an example where non-SI units are still used and relevant to consumers. To make it easier for the consumer, Australia, Canada, New Zealand, and the U.S. use an Energy Star Rating out of 5 stars—the more stars, the more energy efficient the appliance. \(\text{EER}\text{s}\) are expressed in mixed units of British thermal units (Btu) per hour of heating or cooling divided by the power input in watts. Room air conditioners are readily available with \(\text{EER}\text{s}\) ranging from 6 to 12. Although not the same as the \(\text{COP}\text{s}\) just described, these \(\text{EER}\text{s}\) are good for comparison purposes—the greater the \(\text{EER}\), the cheaper an air conditioner is to operate (but the higher its purchase price is likely to be).
The \(\text{EER}\) of an air conditioner or refrigerator can be expressed as
where \({Q}_{\text{c}}\) is the amount of heat transfer from a cold environment in British thermal units, \({t}_{\text{1}}\) is time in hours, \(W\) is the work input in joules, and \({t}_{\text{2}}\) is time in seconds.
- Examine the situation to determine whether heat, work, or internal energy are involved . Look for any system where the primary methods of transferring energy are heat and work. Heat engines, heat pumps, refrigerators, and air conditioners are examples of such systems.
- Identify the system of interest and draw a labeled diagram of the system showing energy flow.
- Identify exactly what needs to be determined in the problem (identify the unknowns) . A written list is useful. Maximum efficiency means a Carnot engine is involved. Efficiency is not the same as the coefficient of performance.
- Make a list of what is given or can be inferred from the problem as stated (identify the knowns) . Be sure to distinguish heat transfer into a system from heat transfer out of the system, as well as work input from work output. In many situations, it is useful to determine the type of process, such as isothermal or adiabatic.
- Solve the appropriate equation for the quantity to be determined (the unknown).
- Substitute the known quantities along with their units into the appropriate equation and obtain numerical solutions complete with units.
- Check the answer to see if it is reasonable: Does it make sense? For example, efficiency is always less than 1, whereas coefficients of performance are greater than 1.
Section Summary
- An artifact of the second law of thermodynamics is the ability to heat an interior space using a heat pump. Heat pumps compress cold ambient air and, in so doing, heat it to room temperature without violation of conservation principles.
- To calculate the heat pump’s coefficient of performance, use the equation \({\text{COP}}_{\text{hp}}=\frac{{Q}_{\text{h}}}{W}\).
- A refrigerator is a heat pump; it takes warm ambient air and expands it to chill it.
Conceptual Questions
Explain why heat pumps do not work as well in very cold climates as they do in milder ones. Is the same true of refrigerators?
In some Northern European nations, homes are being built without heating systems of any type. They are very well insulated and are kept warm by the body heat of the residents. However, when the residents are not at home, it is still warm in these houses. What is a possible explanation?
Why do refrigerators, air conditioners, and heat pumps operate most cost-effectively for cycles with a small difference between \({T}_{\text{h}}\) and \({T}_{\text{c}}\)? (Note that the temperatures of the cycle employed are crucial to its \(\text{COP}\).)
Grocery store managers contend that there is less total energy consumption in the summer if the store is kept at a low temperature. Make arguments to support or refute this claim, taking into account that there are numerous refrigerators and freezers in the store.
Can you cool a kitchen by leaving the refrigerator door open?
Problem Exercises
What is the coefficient of performance of an ideal heat pump that has heat transfer from a cold temperature of \(-\text{25}\text{.}0\text{º}\text{C}\) to a hot temperature of \(\text{40}\text{.}0\text{º}\text{C}\)?
Suppose you have an ideal refrigerator that cools an environment at \(-\text{20}\text{.}0\text{º}\text{C}\) and has heat transfer to another environment at \(\text{50}\text{.}0\text{º}\text{C}\). What is its coefficient of performance?
What is the best coefficient of performance possible for a hypothetical refrigerator that could make liquid nitrogen at \(-\text{200}\text{º}\text{C}\) and has heat transfer to the environment at \(\text{35}\text{.}0\text{º}\text{C}\)?
In a very mild winter climate, a heat pump has heat transfer from an environment at \(5\text{.}\text{00}\text{º}\text{C}\) to one at \(\text{35}\text{.}0\text{º}\text{C}\). What is the best possible coefficient of performance for these temperatures? Explicitly show how you follow the steps in the Problem-Solving Strategies for Thermodynamics .
(a) What is the best coefficient of performance for a heat pump that has a hot reservoir temperature of \(\text{50}\text{.}0\text{º}\text{C}\) and a cold reservoir temperature of \(-\text{20}\text{.0ºC}\)? (b) How much heat transfer occurs into the warm environment if \(3\text{.60}×{\text{10}}^{7}\phantom{\rule{0.25em}{0ex}}\text{J}\) of work (\(\text{10}\text{.}0\text{kW}\cdot \text{h}\)) is put into it? (c) If the cost of this work input is \(\text{10.0 cents/kW}\cdot \text{h}\), how does its cost compare with the direct heat transfer achieved by burning natural gas at a cost of 85.0 cents per therm. (A therm is a common unit of energy for natural gas and equals \(1\text{.}\text{055}×{\text{10}}^{8}\phantom{\rule{0.25em}{0ex}}\text{J}\).)
(b) \(1\text{.}\text{66}×{\text{10}}^{8}\phantom{\rule{0.25em}{0ex}}\text{J}\phantom{\rule{0.25em}{0ex}}\text{or 3}\text{.}\text{97}×{\text{10}}^{4}\phantom{\rule{0.25em}{0ex}}\text{kcal}\)
(c) To transfer \(1\text{.}\text{66}×{\text{10}}^{8}\phantom{\rule{0.25em}{0ex}}\text{J}\) , heat pump costs 💲1.00, natural gas costs 💲1.34.
(a) What is the best coefficient of performance for a refrigerator that cools an environment at \(-\text{30}\text{.}0\text{º}\text{C}\) and has heat transfer to another environment at \(\text{45}\text{.}0º\text{C}\)? (b) How much work in joules must be done for a heat transfer of 4186 kJ from the cold environment? (c) What is the cost of doing this if the work costs 10.0 cents per \(3\text{.}\text{60}×{\text{10}}^{6}\phantom{\rule{0.25em}{0ex}}\text{J}\) (a kilowatt-hour)? (d) How many kJ of heat transfer occurs into the warm environment? (e) Discuss what type of refrigerator might operate between these temperatures.
Suppose you want to operate an ideal refrigerator with a cold temperature of \(-\text{10}\text{.}0º\text{C}\), and you would like it to have a coefficient of performance of 7.00. What is the hot reservoir temperature for such a refrigerator?
\(\text{27.6ºC}\)
An ideal heat pump is being considered for use in heating an environment with a temperature of \(\text{22}\text{.}0\text{º}\text{C}\). What is the cold reservoir temperature if the pump is to have a coefficient of performance of 12.0?
A 4-ton air conditioner removes \(5\text{.}\text{06}×{\text{10}}^{7}\phantom{\rule{0.25em}{0ex}}\text{J}\) (48,000 British thermal units) from a cold environment in 1.00 h. (a) What energy input in joules is necessary to do this if the air conditioner has an energy efficiency rating (\(\text{EER}\)) of 12.0? (b) What is the cost of doing this if the work costs 10.0 cents per \(3\text{.}\text{60}×{\text{10}}^{6}\phantom{\rule{0.25em}{0ex}}\text{J}\) (one kilowatt-hour)? (c) Discuss whether this cost seems realistic. Note that the energy efficiency rating (\(\text{EER}\)) of an air conditioner or refrigerator is defined to be the number of British thermal units of heat transfer from a cold environment per hour divided by the watts of power input.
(a) \(1\text{.}\text{44}×{\text{10}}^{7}\phantom{\rule{0.25em}{0ex}}\text{J}\)
(b) 40 cents
(c) This cost seems quite realistic; it says that running an air conditioner all day would cost 💲9.59 (if it ran continuously).
Show that the coefficients of performance of refrigerators and heat pumps are related by \({\text{COP}}_{\text{ref}}={\text{COP}}_{\text{hp}}-1\).
Start with the definitions of the \(\text{COP}\) s and the conservation of energy relationship between \({Q}_{\text{h}}\), \({Q}_{\text{c}}\), and \(W\) .
Intro to Physics for Non-Majors Copyright © 2012 by OSCRiceUniversity is licensed under a Creative Commons Attribution 4.0 International License , except where otherwise noted.
Share This Book
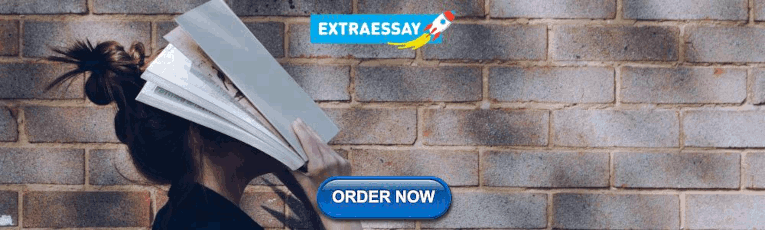
IMAGES
VIDEO
COMMENTS
EXAMPLES SOLUTION. An ideal vapor-compression refrigerant cycle operates at steady state with Refrigerant 134a as the working fluid. Saturated vapor enters the compressor at -100C, and saturated liquid leaves the condenser at 280C. The mass flow rate of refrigerant is 5 kg/min. Determine. The refrigerating capacity, in tons.
Chapter 10: Refrigeration Cycles. The vapor compression refrigeration cycle is a common method for transferring heat from a low temperature to a high temperature. The above figure shows the objectives of refrigerators and heat pumps. The purpose of a refrigerator is the removal of heat, called the cooling load, from a low-temperature medium.
6. constant volume to a final temperature of 425°C, the total entropy increase is 0.4386 kJ/K. The. Answer is (C). 7. Steam enters a turbine with a velocity of 40 m/s and an enthalpy of 3433.8 kJ/kg. At the outlet, 2 meters lower than the inlet, the velocity is 162 m/s, and the enthalpy is 2675.5 kJ/kg. A heat.
2D-1 - Isothermal Vaporization of Water. 2D-2 - Dew Point Calculations for Ammonia. 2D-3 - Volume Occupied by 25 kg of R-134a at Various Temperatures. 2D-4 - Determine Properties Using Thermodynamic Tables. 2D-5 - Relative and Absolute Humidity of Air. 2D-6 - Humidity and Partial Pressure in a Humid Ideal Gas.
In the Carnot engine, which is the most efficient conceivable engine for given source and sink temperature, the efficiency is. η = T2 −T1 T2, (11.8.3) (11.8.3) η = T 2 − T 1 T 2, where T2 and T1 are respectively the temperatures of the hot source and cold sink. If the working substance is taken round a cycle in the PV -plane in the ...
A heat pump uses the same vapour compression refrigeration cycle, see Figure 6.2.1, as a refrigerator. It absorbs heat from a heat sink (e.g., outdoor air in the winter) and delivers (more) heat to a heat source (e.g., indoor air) by consuming work. Applying the first law of thermodynamics to the heat pump cycle, we can derive.
Figure 15.5.3 15.5. 3: A simple heat pump has four basic components: (1) condenser, (2) expansion valve, (3) evaporator, and (4) compressor. In the heating mode, heat transfer Qc Q c occurs to the working fluid in the evaporator (3) from the colder outdoor air, turning it into a gas. The electrically driven compressor (4) increases the ...
In this video, I solved an example of an Ideal Compression Vapor Refrigeration Cycle. Coefficient of Performance is calculated for refrigerator and heat pump.
Refrigeration Cycle Reading Problems 11-1 !11-7, 11-9 11-11, 11-46, 11-49, 11-103 Definitions the 1st law of thermodynamics tells us that heat flow occurs from a hot source to a cooler sink, therefore, energy in the form of work must be added to the process to get heat to flow from a low temperature region to a hot temperature region.
Example Problem 2. Consider a vapor-compression refrigeration cycle that uses R134a as the working fluid. The condenser, which yields saturated liquid, is operated at 45C; the evaporator, which yields saturated vapor, is operated at -10.C. The compressor is 80.% efficient. Using data from the table below, determine:
Figure 12.13 (a) Heat transfer to the gas in a cylinder increases the internal energy of the gas, creating higher pressure and temperature. (b) The force exerted on the movable cylinder does work as the gas expands. Gas pressure and temperature decrease during expansion, indicating that the gas's internal energy has decreased as it does work.
This chapter covers the application of thermodynamics to refrigeration cycles. The first part reviews the first and second laws of thermodynamics and presents methods for calculating thermodynamic properties. ... Solution: (a) Figure 9 shows a schematic p-h diagram for the problem with numerical property data.
Problems chap 11 cen84959_ch11.qxd 6:03 am page 623 chapter 11 refrigeration cycles major application area of thermodynamics is refrigeration, which is the. Skip to document. ... the coefficient of performance of this cascade refrigerator. Solution A cascade refrigeration system operating between the specified pressure limits is considered. ...
Figure 15.28 A simple heat pump has four basic components: (1) condenser, (2) expansion valve, (3) evaporator, and (4) compressor. In the heating mode, heat transfer Q c Q c size 12{Q rSub { size 8{c} } } {} occurs to the working fluid in the evaporator (3) from the colder outdoor air, turning it into a gas. The electrically driven compressor (4) increases the temperature and pressure of the ...
A refrigeration systems produces 150 BTU/lb of cooling. In order to have a rating of 1 ton of refrigeration, what must be the mass flow rate in lb/hr. a. 70 b. 80 c. 90 d. 100. An air compressor is to compress 9m 3 / min from 100kPA to 1 MPa. Assuming ideal conditions and with n = 1, what will be the savings in work due to two-staging? a.
CHAPTER 2 THERMODYNAMICS AND REFRIGERATION CYCLES Thermodynamics is the study of energy, its transformations, and its relation to states of matter. ... Figure 9 shows a schematic p-h diagram for the problem with numerical property data. ... Solution: The mass flow of refrigerant is the same through all components, so it is only computed once ...
As a follow up to Chapter 5, the main focus is about refrigeration and heat pump cycles and their analyses and assessments. The systems considered here are basic vapor-compression refrigeration, air-standard refrigeration, basic heat pumps, cascade refrigeration, and absorption refrigeration. These systems are briefly discussed and multiple ...
PROBLEM-SOLVING STRATEGIES FOR THERMODYNAMICS. Examine the situation to determine whether heat, work, or internal energy are involved. Look for any system where the primary methods of transferring energy are heat and work. Heat engines, heat pumps, refrigerators, and air conditioners are examples of such systems.
Problem Set 3 Thermodynamics and Climate Change MOSTEC 2021 Solutions 1. Concept questions: Answer the following with a brief explanation. (a) For a system undergoing a reversible process: (i) Can there be a total positive change in entropy? (ii) Can there be a total negative change in entropy? (iii) Answer parts (i) and (ii) if instead the ...
Refrigeration Cycle Reading Problems 11-1 → 11-7, 11-9 11-11, 11-44, 11-47, 11-104 Definitions • a refrigeration system removes thermal energy from a low-temperature region and transfers heat to a high-temperature region. • the 1st law of thermodynamics tells us that heat flow occurs from a hot source to a cooler sink, therefore, energy in the form of work must be added to the process ...
When you burn fuel to keep warm, you pay for all of it. The disadvantage is that the work input (required by the second law of thermodynamics) is sometimes more expensive than simply burning fuel, especially if the work is done by electrical energy. The basic components of a heat pump in its heating mode are shown in . A working fluid such as a ...